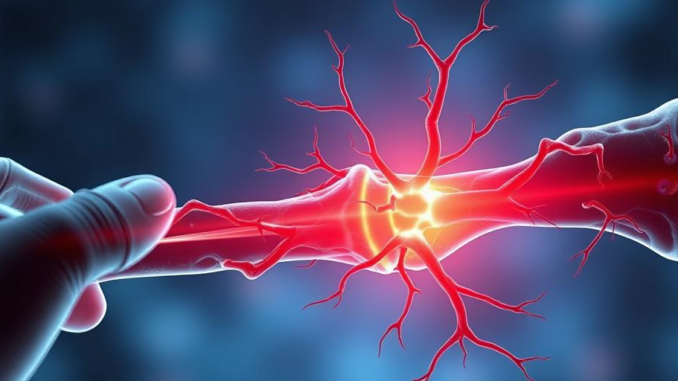
Abstract
Fracture healing is a complex physiological process involving a coordinated cascade of cellular and molecular events. While the general phases of healing—inflammation, soft callus formation, hard callus formation, and remodeling—are well-established, a deeper understanding of the intricate interplay between biomechanical stimuli, vascularization, immune response, and cellular differentiation is crucial for optimizing fracture management and developing novel therapeutic strategies. This review provides a comprehensive overview of the biomechanical and physiological factors influencing fracture healing, highlighting the importance of mechanical stability, angiogenesis, immune cell modulation, and the role of various signaling pathways in regulating bone regeneration. Furthermore, it examines the challenges associated with delayed union and non-union, and explores potential avenues for accelerating and enhancing fracture repair based on a holistic understanding of the biological and mechanical principles involved. The intent is to provide an expert level overview of current understanding in this field, along with the areas where we still require considerable research to ensure we can optimise patient care in the future.
Many thanks to our sponsor Esdebe who helped us prepare this research report.
1. Introduction
Bone fractures represent a significant burden on healthcare systems globally, impacting individuals of all ages and activity levels. Successful fracture healing is paramount for restoring skeletal integrity and function. While many fractures heal uneventfully through a natural regenerative process, a substantial proportion, estimated to be between 5% and 10% depending on fracture type and patient factors, result in delayed union or non-union, requiring further intervention [1, 2]. These complications lead to prolonged morbidity, increased healthcare costs, and a reduced quality of life for affected individuals. Understanding the multifaceted biological and mechanical factors that govern fracture healing is essential for improving clinical outcomes and developing targeted therapies.
The process of fracture healing is remarkably complex, mimicking aspects of embryonic skeletal development and involving the coordinated action of various cell types, growth factors, and signaling pathways [3]. It can be broadly divided into four overlapping phases: (1) inflammation, (2) soft callus formation, (3) hard callus formation, and (4) remodeling. Each phase is characterized by specific cellular and molecular events that contribute to the restoration of bone continuity and structural integrity. This review will delve into the intricate details of these processes, with a particular emphasis on the biomechanical and physiological determinants of fracture healing.
Many thanks to our sponsor Esdebe who helped us prepare this research report.
2. Biomechanical Stimuli in Fracture Healing
Mechanical stimuli play a critical role in regulating fracture healing, influencing cell differentiation, matrix production, and callus remodeling [4]. The concept of “mechanotransduction,” by which cells sense and respond to mechanical forces, is central to understanding this process. Different mechanical environments, such as compression, tension, shear, and fluid flow, exert distinct effects on the healing fracture site [5].
2.1. The Influence of Mechanical Stability:
Optimal mechanical stability is crucial for successful fracture healing. Excessive motion or instability at the fracture site can disrupt callus formation, leading to delayed union or non-union. Studies have demonstrated that stable fixation promotes endochondral ossification and bone bridging, while unstable fixation favors fibrous tissue formation and cartilage persistence [6]. The type of fixation method employed (e.g., cast immobilization, internal fixation) significantly influences the mechanical environment at the fracture site and, consequently, the healing process. The rigidity of the fixation should be carefully considered based on the fracture type, location, and patient factors.
2.2. Role of Interfragmentary Strain:
Interfragmentary strain, defined as the change in length between fracture fragments divided by the original length, is a key determinant of tissue differentiation in the fracture callus. Low strain environments (e.g., compression) promote bone formation, while high strain environments (e.g., tension or shear) favor fibrous tissue or cartilage formation [7]. The optimal strain range for bone healing is believed to be relatively narrow, and deviations from this range can impair the healing process. This is the reason that there is such a focus in the orthopedic community on using optimal fixation techniques that promote compression forces at the fracture site.
2.3. Mechanotransduction Pathways:
Cells within the fracture callus, including mesenchymal stem cells (MSCs), chondrocytes, and osteoblasts, sense mechanical stimuli through various mechanotransduction pathways. These pathways involve a complex interplay of cell surface receptors, intracellular signaling molecules, and gene expression regulators [8]. Key mechanotransduction molecules include integrins, focal adhesion kinase (FAK), Rho GTPases, and ion channels. These molecules mediate the conversion of mechanical signals into biochemical signals that regulate cell proliferation, differentiation, and matrix synthesis. Further research is needed to fully elucidate the intricate details of these pathways and identify potential targets for therapeutic intervention. It is likely that a more complete understanding of these pathways will allow us to fine-tune fracture fixation techniques such that patients can return to full activity as soon as possible.
Many thanks to our sponsor Esdebe who helped us prepare this research report.
3. Vascularization and Angiogenesis
Vascularization is essential for fracture healing, providing oxygen, nutrients, and growth factors to the healing tissue. Angiogenesis, the formation of new blood vessels from pre-existing vasculature, is a critical component of the fracture repair process [9]. Disruption of vascular supply can impair fracture healing and increase the risk of non-union. The vascular response is intricately linked to the mechanical environment, inflammatory signals, and cellular activity at the fracture site.
3.1. Angiogenic Factors:
Several angiogenic factors, including vascular endothelial growth factor (VEGF), fibroblast growth factor-2 (FGF-2), and platelet-derived growth factor (PDGF), play a crucial role in stimulating angiogenesis during fracture healing [10]. These factors are released by inflammatory cells, MSCs, and osteoblasts, and they act on endothelial cells to promote their proliferation, migration, and tube formation. VEGF is considered a master regulator of angiogenesis and is essential for callus vascularization. Disrupting VEGF signaling can significantly impair fracture healing. The development of pro-angiogenic therapies aimed at enhancing vascular supply to the fracture site is an area of active research.
3.2. The Role of Hypoxia:
Hypoxia, or low oxygen tension, is a common feature of the early fracture callus and plays a crucial role in stimulating angiogenesis. Hypoxia-inducible factor-1α (HIF-1α) is a transcription factor that is activated under hypoxic conditions and regulates the expression of VEGF and other angiogenic genes [11]. The hypoxic environment in the fracture callus promotes angiogenesis, but excessive or prolonged hypoxia can impair cell viability and inhibit bone formation. The optimal balance between hypoxia and oxygenation is critical for successful fracture healing. We are beginning to understand that the correct balance of oxygen tension is essential to ensure appropriate bone healing, it is likely that this varies from patient to patient. The future of this field is likely to be a patient by patient approach that is able to predict the healing response.
3.3. Microvascular Architecture:
The microvascular architecture of the fracture callus is highly dynamic and adapts to the changing mechanical and physiological environment. The early callus is characterized by a dense network of leaky blood vessels that deliver oxygen and nutrients to the proliferating cells. As the callus matures, the microvasculature becomes more organized and specialized, with distinct regions of bone formation and remodeling [12]. Impaired microvascular remodeling can contribute to delayed union and non-union. Further research is needed to understand the complex interplay between angiogenesis, microvascular architecture, and bone formation during fracture healing.
Many thanks to our sponsor Esdebe who helped us prepare this research report.
4. Inflammatory Response and Immune Cell Modulation
The inflammatory response is an essential component of fracture healing, initiating the repair process and recruiting immune cells to the fracture site. However, excessive or prolonged inflammation can impair healing and lead to complications. Modulating the inflammatory response and immune cell activity is crucial for optimizing fracture repair [13].
4.1. Inflammatory Cytokines:
A cascade of inflammatory cytokines, including tumor necrosis factor-α (TNF-α), interleukin-1β (IL-1β), and interleukin-6 (IL-6), are released at the fracture site, initiating the inflammatory response [14]. These cytokines recruit neutrophils, macrophages, and other immune cells to the fracture site, where they clear debris, release growth factors, and stimulate angiogenesis. However, excessive production of inflammatory cytokines can inhibit osteoblast differentiation and bone formation. Balancing the pro-inflammatory and anti-inflammatory responses is crucial for successful fracture healing.
4.2. Macrophage Polarization:
Macrophages are key immune cells that play a critical role in regulating fracture healing. Macrophages can be polarized into two main phenotypes: M1 macrophages, which are pro-inflammatory and promote tissue degradation, and M2 macrophages, which are anti-inflammatory and promote tissue repair [15]. The balance between M1 and M2 macrophages is critical for successful fracture healing. Shifting the macrophage polarization towards the M2 phenotype can accelerate fracture repair. Therapeutic strategies aimed at modulating macrophage polarization are being investigated as potential approaches to enhance fracture healing. Currently, this is difficult to achieve, however with the continued improvement of our understanding of cell signaling pathways it is feasible that we will be able to achieve this in the future.
4.3. The Role of T Cells:
T cells, another type of immune cell, also play a role in regulating fracture healing. T helper cells (Th cells) can be divided into different subsets, including Th1 cells, which promote inflammation, and Th2 cells, which promote tissue repair. The balance between Th1 and Th2 cells can influence the outcome of fracture healing [16]. Regulatory T cells (Tregs) are a subset of T cells that suppress the immune response and promote tissue repair. Tregs have been shown to promote fracture healing by suppressing inflammation and stimulating osteoblast differentiation. The manipulation of T cell subsets may hold promise for improving fracture repair.
Many thanks to our sponsor Esdebe who helped us prepare this research report.
5. Cellular Differentiation and Bone Formation
The differentiation of MSCs into chondrocytes and osteoblasts is a critical step in fracture healing. This process is regulated by a complex interplay of growth factors, signaling pathways, and mechanical stimuli [17]. Understanding the mechanisms that govern cellular differentiation is essential for developing strategies to enhance bone formation.
5.1. Growth Factors:
Several growth factors, including bone morphogenetic proteins (BMPs), transforming growth factor-β (TGF-β), and insulin-like growth factor-1 (IGF-1), play a crucial role in regulating MSC differentiation and bone formation [18]. BMPs are potent osteoinductive factors that stimulate osteoblast differentiation and bone formation. TGF-β promotes chondrogenesis and cartilage formation, while IGF-1 stimulates osteoblast proliferation and matrix synthesis. The delivery of growth factors to the fracture site can accelerate bone healing, but the optimal dose and delivery method remain a subject of ongoing research. BMP2 is commonly used clinically to improve bone healing, however there are known complications from its use. It is likely that in the future we will have a range of factors that are used depending on the individual patient needs.
5.2. Signaling Pathways:
Several signaling pathways, including the Wnt, Hedgehog, and Notch pathways, are involved in regulating MSC differentiation and bone formation [19]. The Wnt pathway promotes osteoblast differentiation and bone formation, while the Hedgehog pathway regulates chondrocyte differentiation and cartilage formation. The Notch pathway plays a role in cell fate decisions and tissue patterning. Dysregulation of these signaling pathways can impair fracture healing. Targeting these signaling pathways may hold promise for improving bone regeneration.
5.3. Matrix Remodeling:
Matrix remodeling is an essential process for bone formation and fracture healing. Enzymes called matrix metalloproteinases (MMPs) degrade and remodel the extracellular matrix, allowing for cell migration and tissue regeneration [20]. The balance between MMP activity and tissue inhibitors of metalloproteinases (TIMPs) is critical for successful fracture healing. Excessive MMP activity can lead to cartilage degradation and impaired bone formation. Inhibiting MMP activity may promote bone healing in certain situations. Further understanding of the complex interplay between matrix remodeling and bone formation is needed to develop targeted therapies.
Many thanks to our sponsor Esdebe who helped us prepare this research report.
6. Challenges in Fracture Healing: Delayed Union and Non-Union
Delayed union and non-union represent significant clinical challenges, often requiring further surgical intervention. These complications are characterized by impaired callus formation, persistent inflammation, and inadequate vascularization [21]. Understanding the underlying causes of delayed union and non-union is essential for developing effective treatment strategies.
6.1. Risk Factors:
Several risk factors can contribute to delayed union and non-union, including fracture severity, patient age, smoking, diabetes, and certain medications [22]. Open fractures, which involve disruption of the surrounding soft tissues and contamination of the fracture site, are at higher risk of complications. Older patients have a reduced capacity for bone regeneration, while smoking and diabetes impair vascularization and cellular function. Certain medications, such as nonsteroidal anti-inflammatory drugs (NSAIDs) and corticosteroids, can inhibit bone healing. Identifying and managing these risk factors is crucial for preventing delayed union and non-union.
6.2. Biological Mechanisms:
The biological mechanisms underlying delayed union and non-union are complex and multifactorial. Impaired vascularization, persistent inflammation, and inadequate MSC differentiation are common features of these complications [23]. The fracture site may be deficient in growth factors and signaling molecules necessary for bone formation. In addition, excessive mechanical instability can disrupt callus formation and lead to fibrous tissue formation. A thorough understanding of the biological mechanisms involved is essential for developing targeted therapies to promote bone healing.
6.3. Treatment Strategies:
Treatment strategies for delayed union and non-union typically involve surgical intervention to improve mechanical stability and promote bone regeneration. Bone grafting, which involves transplanting bone tissue from another site in the body or using synthetic bone substitutes, is often used to stimulate bone formation [24]. Growth factor delivery, such as BMPs, can also be used to enhance bone healing. In some cases, electrical stimulation or ultrasound therapy may be used to stimulate bone regeneration. The choice of treatment strategy depends on the specific characteristics of the fracture and the patient’s overall health. These treatments have variable success rates and further research is required to optimise the approaches.
Many thanks to our sponsor Esdebe who helped us prepare this research report.
7. Future Directions and Therapeutic Strategies
Future research efforts should focus on developing novel therapeutic strategies to accelerate and enhance fracture healing. This includes the development of biomaterials that mimic the natural bone matrix, the delivery of growth factors and signaling molecules to the fracture site, and the modulation of the immune response to promote tissue repair [25].
7.1. Biomaterials:
Biomaterials play a crucial role in fracture healing by providing a scaffold for cell attachment and tissue regeneration. Ideal biomaterials should be biocompatible, biodegradable, and osteoconductive, meaning that they promote bone ingrowth [26]. Examples of biomaterials used in fracture healing include calcium phosphate ceramics, collagen, and synthetic polymers. The development of biomaterials that release growth factors or signaling molecules can further enhance bone regeneration. Advances in nanotechnology and 3D printing are enabling the creation of more sophisticated biomaterials with tailored properties to promote fracture healing.
7.2. Gene Therapy:
Gene therapy holds promise for enhancing fracture healing by delivering genes that encode growth factors or signaling molecules to the fracture site [27]. Viral vectors, such as adeno-associated virus (AAV), can be used to deliver genes to cells in the fracture callus. Gene therapy can be used to stimulate osteoblast differentiation, angiogenesis, and matrix synthesis. However, safety concerns and regulatory hurdles need to be addressed before gene therapy can be widely used in clinical practice.
7.3. Cell-Based Therapies:
Cell-based therapies, such as MSC transplantation, can be used to enhance fracture healing by providing a source of osteogenic cells to the fracture site [28]. MSCs can be harvested from bone marrow, adipose tissue, or other sources and expanded in vitro before being transplanted into the fracture site. MSCs can differentiate into osteoblasts and secrete growth factors that promote bone formation. Cell-based therapies have shown promise in preclinical studies, but more clinical trials are needed to evaluate their efficacy and safety. One of the key issues is the lack of standard approaches for cell harvesting, expansion and introduction into the patient.
7.4. Personalized Medicine:
The future of fracture management lies in personalized medicine, where treatment strategies are tailored to the individual patient based on their genetic profile, medical history, and fracture characteristics [29]. This approach takes into account the variability in bone healing rates and outcomes among individuals. Biomarkers that predict fracture healing potential can be used to identify patients who are at high risk of delayed union or non-union. Personalized treatment strategies may involve the use of targeted therapies that address the specific biological deficits in each patient. Further research is needed to identify and validate biomarkers that can predict fracture healing outcomes and guide treatment decisions. It is likely that the future of fracture treatment will involve AI approaches to help predict and optimise the patient pathways.
Many thanks to our sponsor Esdebe who helped us prepare this research report.
8. Conclusion
Fracture healing is a complex and dynamic process that is influenced by a multitude of biological and mechanical factors. Understanding the intricate interplay between these factors is essential for optimizing fracture management and developing novel therapeutic strategies. Mechanical stability, vascularization, immune cell modulation, and cellular differentiation are all critical components of successful fracture healing. While significant progress has been made in understanding the mechanisms of fracture repair, further research is needed to address the challenges associated with delayed union and non-union. Future efforts should focus on developing personalized treatment strategies that are tailored to the individual patient based on their genetic profile, medical history, and fracture characteristics. The integration of new technologies, such as biomaterials, gene therapy, and cell-based therapies, holds promise for accelerating and enhancing fracture healing, ultimately improving patient outcomes and reducing the burden of fractures on healthcare systems.
Many thanks to our sponsor Esdebe who helped us prepare this research report.
References
[1] Bhandari, M., et al. “A prospective study of the incidence of nonunion after intramedullary nailing of tibial shaft fractures.” Journal of Orthopaedic Trauma 17.7 (2003): 467-471.
[2] Zura, R., et al. “Definitions of delayed union and nonunion: a Delphi study.” Journal of Bone and Joint Surgery 93.2 (2011): 113-120.
[3] Einhorn, T. A., and M. Gerstenfeld. “Fracture healing: mechanisms and interventions.” Journal of the American Academy of Orthopaedic Surgeons 23.5 (2015): 343-348.
[4] Claes, L., et al. “Mechanical stimulation of bone regeneration: molecular and cellular mechanisms.” Clinical Orthopaedics and Related Research 355S (1998): S132-S142.
[5] Carter, D. R., et al. “Mechanical loading history and skeletal biology.” Journal of Biomechanics 31.1 (1998): 1-9.
[6] Perren, S. M. “Physical and biological aspects of fracture healing with special reference to internal fixation.” Clinical Orthopaedics and Related Research 138 (1979): 175-196.
[7] Prendergast, P. J., et al. “Computational biomechanics in fracture healing.” Philosophical Transactions of the Royal Society B: Biological Sciences 361.1470 (2006): 1441-1447.
[8] Khan, S. N., et al. “Mechanotransduction pathways in bone: the role of integrins.” Journal of Bone and Joint Surgery 90-A.3 (2008): 636-644.
[9] Marsell, R., and E. B. Einhorn. “The biology of fracture healing.” Injury 42.6 (2011): 551-555.
[10] Street, J., et al. “Vascular endothelial growth factor stimulates bone repair by promoting angiogenesis and osteoblast differentiation.” Journal of Bone and Mineral Research 17.12 (2002): 2249-2258.
[11] Hu, Y., et al. “Hypoxia-inducible factor-1α and fracture healing.” Journal of Orthopaedic Research 27.12 (2009): 1528-1535.
[12] Hassan, M. U., et al. “Angiogenesis and vascular remodeling during bone fracture healing.” Journal of Cellular Physiology 229.1 (2014): 1-8.
[13] Mountziaris, P. M., and A. G. Mikos. “Biomaterial-induced inflammation: a paradox for tissue engineering.” Biomaterials 29.16 (2008): 2589-2595.
[14] Gerstenfeld, L. C., et al. “Expression of cytokines during fracture healing.” Journal of Bone and Mineral Research 18.3 (2003): 516-527.
[15] Loi, F., et al. “Fracture healing: Injury repair and regeneration.” Nature Reviews Materials 6 (2021): 99-111.
[16] Yang, F., et al. “The immune microenvironment in fracture healing: a systematic review.” Journal of Orthopaedic Research 39.3 (2021): 475-488.
[17] Bianco, P., et al. “Mesenchymal stem cells: revisiting history, concepts, and assays.” Cell Stem Cell 2.4 (2008): 313-319.
[18] Canalis, E., et al. “Growth factors and cytokines in bone cell metabolism.” Endocrine Reviews 24.2 (2003): 155-173.
[19] Baron, R., and G. Rawadi. “Targeting the Wnt/β-catenin pathway to regulate bone repair.” Bone 43.4 (2008): 631-646.
[20] Holmbeck, K., et al. “MT1-MMP: a key regulator of matrix remodeling during development and disease.” Trends in Cell Biology 14.12 (2004): 639-647.
[21] Calori, G. M., et al. “Treatment of non-union and delayed union in long bones.” Injury 38.4 (2007): 393-407.
[22] Schmidt, A. H. “Nonunion of the tibia: risk factors, evaluation, and treatment options.” Journal of the American Academy of Orthopaedic Surgeons 17.9 (2009): 556-567.
[23] Dimitriou, R., et al. “The role of local factors on non-union.” Injury 36S4 (2005): S31-S39.
[24] Giannoudis, P. V., et al. “Bone substitutes: an update.” Injury 42S2 (2011): S2-S16.
[25] Bose, S., et al. “Bone tissue engineering scaffolds from novel compositions and architectures.” Biomaterials 33.11 (2012): 2785-2804.
[26] Jones, J. R. “Review of bioactive glass: from Hench to hybrids.” Acta Biomaterialia 9.1 (2013): 1-23.
[27] Evans, C. H., and P. D. Robbins. “Gene therapy for arthritis.” Gene Therapy 18.1 (2011): 3-11.
[28] Caplan, A. I. “Mesenchymal stem cells.” Journal of Orthopaedic Research 9.5 (1991): 641-650.
[29] Edwards, J. R., et al. “Biomarkers of bone formation and resorption as diagnostic indicators of bone fracture healing.” JBMR Plus 3.1 (2019): e10139.
So, if I understand correctly, the future of fracture healing involves less “hammer it ’til it fits” and more “bespoke bone boutiques” tailoring treatments to our individual genetic predispositions? I’m picturing tiny orthopaedic tailors measuring our DNA instead of inseams. Very chic!
That’s a fantastic analogy! The vision of “bespoke bone boutiques” is certainly where we’re headed. Imagine leveraging AI to analyze individual patient data and create personalized treatment plans. It’s about precision, not just brute force, to optimize healing. Thanks for sparking that image!
Editor: MedTechNews.Uk
Thank you to our Sponsor Esdebe
The discussion around mechanotransduction pathways is fascinating. Exploring how we can translate that knowledge into truly optimized, patient-specific fixation techniques seems key to accelerating recovery and improving long-term outcomes.