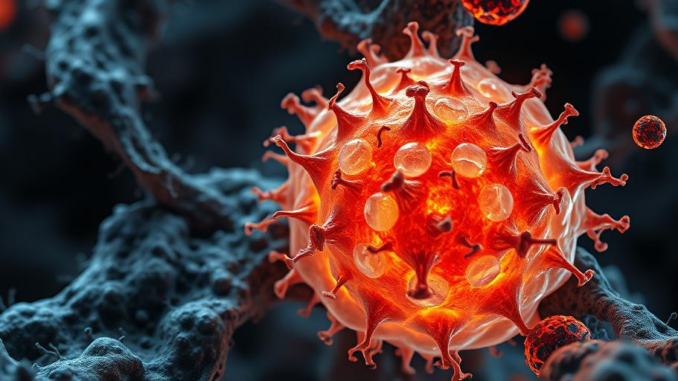
Abstract
Tumor heterogeneity, a complex interplay of genetic, epigenetic, and microenvironmental factors, poses a significant challenge to effective cancer treatment. This report delves into the multifaceted aspects of tumor heterogeneity, examining its origins, manifestations, and implications for therapeutic resistance. We explore the latest research on intratumoral and intertumoral heterogeneity, focusing on the role of the tumor microenvironment (TME) in shaping tumor evolution and influencing treatment response. Furthermore, we discuss emerging strategies for characterizing and targeting tumor heterogeneity, including advanced imaging techniques, single-cell analysis, and personalized treatment approaches. The convergence of these approaches holds promise for improving patient outcomes by addressing the inherent complexity of cancer.
Many thanks to our sponsor Esdebe who helped us prepare this research report.
1. Introduction
Cancer, fundamentally a disease of uncontrolled cell growth, is far from a monolithic entity. The notion of a singular ‘cancer’ has given way to a more nuanced understanding of tumor biology, highlighting the profound impact of tumor heterogeneity. This heterogeneity manifests at multiple levels, from genetic mutations and epigenetic modifications within individual tumor cells to variations in the composition and organization of the tumor microenvironment (TME) [1]. Understanding the origins and consequences of this heterogeneity is crucial for developing effective and durable cancer therapies.
Intratumoral heterogeneity refers to the diversity of cancer cells within a single tumor mass. These cells can differ in their genetic makeup, gene expression profiles, and phenotypic characteristics, leading to variations in growth rate, metastatic potential, and sensitivity to therapy. Intertumoral heterogeneity, on the other hand, describes the differences observed between tumors arising in different patients with the same cancer type. This can be attributed to variations in germline genetics, environmental exposures, and the specific mutations driving tumor development [2].
The tumor microenvironment (TME), a complex ecosystem surrounding cancer cells, plays a critical role in shaping tumor heterogeneity. The TME comprises a diverse array of cells, including fibroblasts, immune cells, endothelial cells, and pericytes, as well as extracellular matrix (ECM) components, growth factors, cytokines, and chemokines. These components interact with cancer cells in a dynamic and reciprocal manner, influencing tumor growth, angiogenesis, immune evasion, and metastatic dissemination [3].
This report aims to provide a comprehensive overview of tumor heterogeneity, exploring its underlying mechanisms, its impact on treatment response, and the emerging strategies for overcoming the challenges it presents. We will discuss the role of genetic and epigenetic alterations, the influence of the TME, and the latest advancements in imaging and molecular profiling technologies that are enabling a more personalized approach to cancer therapy.
Many thanks to our sponsor Esdebe who helped us prepare this research report.
2. Genetic and Epigenetic Origins of Tumor Heterogeneity
The genetic landscape of tumors is characterized by a diverse array of mutations, including point mutations, insertions, deletions, copy number alterations, and chromosomal rearrangements. These mutations can arise spontaneously during DNA replication or be induced by environmental factors such as radiation and carcinogens. The accumulation of these mutations over time can lead to the emergence of subclones within a tumor, each with a distinct genetic profile [4].
Not all mutations are created equal. Driver mutations are those that confer a selective growth advantage to cancer cells, promoting tumor development and progression. Passenger mutations, on the other hand, do not directly contribute to cancer growth but may be associated with other mutations that do [5]. The identification of driver mutations is crucial for developing targeted therapies that specifically inhibit the oncogenic pathways driving tumor growth.
Epigenetic modifications, such as DNA methylation and histone modifications, also play a significant role in tumor heterogeneity. These modifications can alter gene expression without changing the underlying DNA sequence, leading to variations in cellular phenotype and behavior. Epigenetic alterations can be influenced by both genetic and environmental factors, and they can be reversible, offering potential therapeutic targets [6].
Furthermore, the concept of cancer stem cells (CSCs) contributes significantly to tumor heterogeneity. CSCs are a small subpopulation of tumor cells that possess stem cell-like properties, including the ability to self-renew and differentiate into other cell types within the tumor. CSCs are thought to be responsible for tumor initiation, metastasis, and resistance to therapy [7]. The identification and targeting of CSCs is a major focus of cancer research.
Many thanks to our sponsor Esdebe who helped us prepare this research report.
3. The Role of the Tumor Microenvironment in Shaping Heterogeneity
The tumor microenvironment (TME) is a complex and dynamic ecosystem that profoundly influences tumor behavior. It is composed of a diverse array of cells, including fibroblasts, immune cells, endothelial cells, and pericytes, as well as extracellular matrix (ECM) components, growth factors, cytokines, and chemokines [8]. These components interact with cancer cells in a reciprocal manner, influencing tumor growth, angiogenesis, immune evasion, and metastatic dissemination.
Cancer-associated fibroblasts (CAFs) are a major component of the TME and play a critical role in tumor progression. CAFs secrete growth factors, cytokines, and ECM components that promote tumor cell proliferation, angiogenesis, and invasion. They can also remodel the ECM, creating a more permissive environment for tumor cell migration [9].
Immune cells, both innate and adaptive, also play a complex and often contradictory role in the TME. While some immune cells, such as cytotoxic T lymphocytes (CTLs) and natural killer (NK) cells, can directly kill cancer cells, others, such as myeloid-derived suppressor cells (MDSCs) and tumor-associated macrophages (TAMs), can suppress anti-tumor immunity and promote tumor growth [10]. The balance between these pro- and anti-tumor immune responses determines the overall outcome of the immune response to cancer.
Angiogenesis, the formation of new blood vessels, is essential for tumor growth and metastasis. Tumors secrete angiogenic factors, such as vascular endothelial growth factor (VEGF), which stimulate endothelial cells to proliferate and form new blood vessels. These new blood vessels provide tumors with the nutrients and oxygen they need to grow, and they also provide a route for cancer cells to escape the primary tumor and metastasize to distant sites [11].
The ECM, a complex network of proteins and polysaccharides, provides structural support to the TME and plays a critical role in cell-cell and cell-matrix interactions. The ECM can be remodeled by cancer cells and stromal cells, leading to changes in its composition and organization. These changes can influence tumor cell adhesion, migration, and invasion [12].
Many thanks to our sponsor Esdebe who helped us prepare this research report.
4. Imaging Techniques for Characterizing Tumor Heterogeneity
Advanced imaging techniques are essential for visualizing and characterizing tumor heterogeneity in vivo. These techniques can provide information about tumor size, shape, location, and vascularity, as well as the distribution of different cell types within the tumor [13].
Magnetic resonance imaging (MRI) is a non-invasive imaging technique that provides high-resolution images of soft tissues. MRI can be used to detect and characterize tumors in various organs, including the brain, breast, and prostate. Functional MRI (fMRI) can be used to assess tumor blood flow and oxygenation [14].
Computed tomography (CT) is another non-invasive imaging technique that uses X-rays to create cross-sectional images of the body. CT is particularly useful for detecting and characterizing tumors in the lungs, liver, and bones. Positron emission tomography (PET) is a nuclear medicine imaging technique that uses radioactive tracers to detect and visualize metabolic activity in the body. PET can be used to detect and stage tumors, as well as to monitor treatment response [15]. Combining PET with CT (PET/CT) provides both anatomical and functional information about tumors.
Optical imaging techniques, such as fluorescence microscopy and bioluminescence imaging, are widely used in preclinical research to study tumor heterogeneity in vivo. These techniques can be used to visualize the expression of specific proteins or genes within tumors, as well as to track the movement of cancer cells [16].
Ultrasound imaging is a non-invasive and inexpensive imaging technique that uses sound waves to create images of soft tissues. Ultrasound can be used to detect and characterize tumors in the breast, liver, and thyroid. Contrast-enhanced ultrasound can improve the sensitivity and specificity of ultrasound imaging [17].
More recently, advanced microscopy techniques such as multiphoton microscopy and intravital microscopy are being used to visualize the TME in real-time, providing valuable insights into the dynamic interactions between cancer cells and stromal cells [18]. These techniques are enabling researchers to study tumor heterogeneity at a cellular and molecular level.
Many thanks to our sponsor Esdebe who helped us prepare this research report.
5. Personalized Treatment Approaches Based on Tumor Genetics and Microenvironment
The increasing understanding of tumor heterogeneity has led to the development of personalized treatment approaches that are tailored to the individual characteristics of each patient’s tumor. These approaches take into account the genetic and epigenetic profile of the tumor, as well as the composition and organization of the TME [19].
Genomic sequencing is now routinely used to identify driver mutations in tumors. This information can be used to select targeted therapies that specifically inhibit the oncogenic pathways driving tumor growth. For example, patients with tumors harboring mutations in the EGFR gene may benefit from treatment with EGFR inhibitors [20].
Immunotherapy, which harnesses the power of the immune system to fight cancer, has emerged as a promising treatment approach for many types of cancer. However, not all patients respond to immunotherapy. Biomarkers, such as PD-L1 expression and tumor mutational burden (TMB), can be used to predict which patients are most likely to benefit from immunotherapy [21].
Targeting the TME is another promising approach to overcoming tumor heterogeneity. Strategies include inhibiting angiogenesis, disrupting CAF activity, and modulating the immune response within the TME. For example, anti-angiogenic therapies, such as bevacizumab, can inhibit VEGF signaling and reduce tumor blood flow [22].
Single-cell analysis techniques, such as single-cell RNA sequencing (scRNA-seq), are providing unprecedented insights into tumor heterogeneity. These techniques can be used to identify distinct subpopulations of cancer cells within a tumor, as well as to characterize the gene expression profiles of individual cells in the TME. This information can be used to develop more targeted and effective therapies [23].
Adaptive therapy is a novel treatment approach that aims to control tumor growth by dynamically adjusting drug dosages based on real-time monitoring of tumor response. The goal of adaptive therapy is to maintain a stable disease state rather than eradicating the tumor completely, which can lead to the emergence of resistance [24].
Many thanks to our sponsor Esdebe who helped us prepare this research report.
6. Overcoming Therapeutic Resistance in Heterogeneous Tumors
Therapeutic resistance remains a major challenge in cancer treatment. Tumor heterogeneity plays a significant role in the development of resistance, as subpopulations of cancer cells may be inherently resistant to specific therapies or may acquire resistance over time [25].
Several mechanisms can contribute to therapeutic resistance, including:
- Genetic mutations: Cancer cells can acquire mutations that confer resistance to specific drugs. For example, mutations in the EGFR gene can lead to resistance to EGFR inhibitors.
- Epigenetic modifications: Epigenetic changes can alter gene expression and lead to resistance to therapy.
- Activation of alternative signaling pathways: Cancer cells can activate alternative signaling pathways that bypass the inhibited pathway, leading to resistance.
- Changes in drug metabolism: Cancer cells can alter their metabolism to reduce the effectiveness of drugs.
- Increased drug efflux: Cancer cells can increase the expression of drug efflux pumps, which pump drugs out of the cell, reducing their intracellular concentration.
- Alterations in the TME: The TME can protect cancer cells from therapy and promote resistance.
- Phenotypic plasticity: Cancer cells can switch between different phenotypes, some of which may be more resistant to therapy.
To overcome therapeutic resistance, it is essential to develop strategies that target multiple pathways simultaneously and that account for the heterogeneity of the tumor. Combination therapies, which combine two or more drugs with different mechanisms of action, can be more effective than single-agent therapies [26].
Another approach is to use drugs that target the TME, such as anti-angiogenic therapies or drugs that modulate the immune response. These drugs can make cancer cells more susceptible to chemotherapy or radiation therapy [27].
Finally, adaptive therapy can be used to control tumor growth and prevent the emergence of resistance. By dynamically adjusting drug dosages based on real-time monitoring of tumor response, adaptive therapy can maintain a stable disease state and prolong survival [24].
Many thanks to our sponsor Esdebe who helped us prepare this research report.
7. Future Directions and Concluding Remarks
Tumor heterogeneity remains a formidable challenge in cancer research and treatment. However, the increasing understanding of the genetic, epigenetic, and microenvironmental factors that contribute to heterogeneity is paving the way for more personalized and effective therapies. Future research should focus on developing more sophisticated imaging techniques for characterizing tumor heterogeneity in vivo, as well as on developing new drugs that target multiple pathways simultaneously and that account for the heterogeneity of the tumor.
Furthermore, there is a growing need for improved methods for analyzing and integrating the vast amounts of data generated by genomic sequencing, single-cell analysis, and imaging studies. Artificial intelligence (AI) and machine learning (ML) are emerging as powerful tools for analyzing complex datasets and identifying patterns that would be difficult or impossible to detect manually. AI and ML can be used to predict treatment response, identify novel drug targets, and develop personalized treatment strategies [28].
In conclusion, addressing tumor heterogeneity is paramount for improving cancer outcomes. The convergence of advanced imaging, molecular profiling, and computational approaches holds immense promise for unraveling the complexities of tumor biology and developing more effective and durable cancer therapies. While significant challenges remain, the progress made in recent years provides a foundation for continued innovation and ultimately, a brighter future for cancer patients.
Many thanks to our sponsor Esdebe who helped us prepare this research report.
References
[1] McGranahan, N., & Swanton, C. (2017). Clonal heterogeneity and tumor evolution: past, present, and the future. Cell, 168(4), 613-628.
[2] Vogelstein, B., Papadopoulos, N., Velculescu, V. E., Zhou, S., Diaz Jr, L. A., & Kinzler, K. W. (2013). Cancer genome landscapes. Science, 339(6127), 1546-1558.
[3] Hanahan, D., & Weinberg, R. A. (2011). Hallmarks of cancer: the next generation. Cell, 144(5), 646-674.
[4] Greaves, M., & Maley, C. C. (2012). Clonal evolution in cancer. Nature, 481(7381), 306-313.
[5] Bozic, I., Allen, B., Nowak, M. A., & Vogelstein, B. (2010). Evolutionary dynamics of cancer. Annual review of biomedical engineering, 12, 269-291.
[6] Dawson, M. A., & Kouzarides, T. (2012). Cancer epigenetics: from mechanism to therapy. Cell, 150(1), 12-27.
[7] Clarke, M. F., Dick, J. E., Dirks, P. B., Eaves, C. J., Jamieson, C. H., Jones, D. L., … & Passegué, E. (2006). Cancer stem cells—perspectives on current status and future directions: AACR Workshop on cancer stem cells. Cancer research, 66(19), 9339-9344.
[8] Quail, D. F., & Joyce, J. A. (2017). Microenvironmental regulation of tumor progression and metastasis. Nature medicine, 19(11), 1423-1437.
[9] Kalluri, R. (2016). The biology and function of fibroblasts in cancer. Nature Reviews Cancer, 16(9), 582-598.
[10] Coussens, L. M., Zitvogel, L., & Palucka, A. K. (2013). Neutralizing tumor-promoting chronic inflammation: a magic bullet?. Science, 339(6117), 286-291.
[11] Carmeliet, P. (2005). Angiogenesis in cancer and other diseases. Nature, 438(7070), 932-936.
[12] Lu, P., Weaver, V. M., & Werb, Z. (2012). The extracellular matrix: a dynamic niche in cancer progression. Journal of Cell Biology, 196(4), 395-406.
[13] Gerstner, E. R., & Batchelor, T. T. (2010). Improving outcomes in glioblastoma: the role of imaging. Journal of Clinical Oncology, 28(3), 487-496.
[14] Gillies, R. J., Verduzco, D., Gatenby, R. A. (2012). Evolutionary dynamics of carcinogenesis. Nature Reviews Cancer, 12, 47-61.
[15] Lodge, M. A. (2009). Image quantitation in PET: basic principles. Seminars in Nuclear Medicine, 39(4), 219-239.
[16] Weissleder, R. (2001). Scaling down imaging: molecular mapping of cancer in mice. Nature Reviews Cancer, 2(1), 11-18.
[17] Sidhu, P. S., & Dudea, S. M. (2018). Contrast-enhanced ultrasound of focal liver lesions. World Journal of Gastroenterology, 24(28), 3097-3121.
[18] Ritsma, L., Steller, E. J., Beerling, E., Loomans, C. J., Zwart, W., Van Rheenen, J. (2012). Intravital microscopy reveals reversible multi-cellular cooperation during melanoma invasion. Proceedings of the National Academy of Sciences, 109, 290-295.
[19] Garralda, E., Dienstmann, R., Tabernero, J. (2013). Biomarkers and molecular targets in cancer: from conventional approaches to promising novel strategies. Oncology, 85, 66-77.
[20] Sharma, S. V., Bell, D. W., Settleman, J., Haber, D. A. (2007). Epidermal growth factor receptor mutations in lung cancer. Nature Reviews Cancer, 7, 169-181.
[21] Topalian, S. L., Hodi, F. S., Brahmer, J. R., Gettinger, S. N., Smith, D. C., McDermott, D. F., … & Pardoll, D. M. (2012). Safety, activity, and immune correlates of anti-PD-1 antibody in cancer. New England Journal of Medicine, 366(26), 2443-2454.
[22] Ferrara, N., Hillan, K. J., & Novotny, W. (2005). Bevacizumab (Avastin), an anti-VEGF humanized monoclonal antibody for cancer therapy. Biochemical and biophysical research communications, 333(2), 328-335.
[23] Patel, A. P., Tirosh, I., Trombetta, J. J., Shalek, A. K., Reynolds, S. M., Miller, A. K., … & Regev, A. (2014). Single-cell RNA-seq highlights intratumoral heterogeneity in primary glioblastoma. Science, 344(6190), 1396-1401.
[24] Gatenby, R. A., Silva, A. S., Gillies, R. J., & Frieden, B. R. (2009). Adaptive therapy. Cancer research, 69(11), 4894-4903.
[25] Holohan, C., van Schaeybroeck, S., Longley, D. B., & Johnston, P. G. (2013). Cancer drug resistance: an evolving paradigm. Nature Reviews Cancer, 13(10), 714-726.
[26] Bayat Mokhtari, R., Kumar, A., Ghasemi, Y., Rezazadeh, H., Mehrzadi, S., Barar, J., & Omidi, Y. (2017). Combination therapy in combating cancer. Oncotarget, 8(20), 38022.
[27] De Palma, M., & Hanahan, D. (2012). The biology of personalized cancer medicine: targeting the microenvironment. Cell, 148(4), 637-652.
[28] Esteva, A., Kuprel, B., Novoa, R. A., Ko, J., Swani, S. M., Blau, H. M., … & Threlfall, C. J. (2017). Dermatologist-level classification of skin cancer with deep neural networks. Nature, 542(7639), 115-118.
Be the first to comment