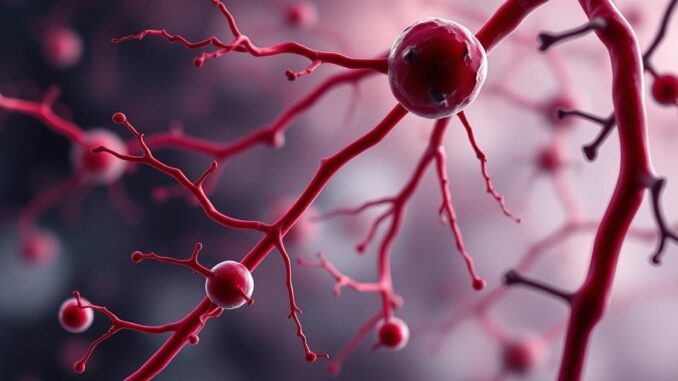
Abstract
Blood vessels, the circulatory system’s lifeline, are crucial for delivering oxygen, nutrients, and immune cells throughout the body. Their intricate network and dynamic behavior are essential for tissue homeostasis and repair. This research report delves into the multifaceted aspects of blood vessel biology, encompassing developmental angiogenesis, the complexities of mature vessel function, and the therapeutic potential of angiogenesis and anti-angiogenesis strategies. We will explore the molecular mechanisms driving blood vessel formation, the influence of the microenvironment, and the latest advancements in engineering functional vascular networks. Special attention will be given to the emerging field of engineered blood vessel cells (R-VECs) and their role in enhancing the success of islet transplantation and other regenerative medicine applications. Furthermore, we will discuss the challenges associated with creating stable and functional blood vessel networks in vivo and explore future directions for research and clinical translation.
Many thanks to our sponsor Esdebe who helped us prepare this research report.
1. Introduction
The circulatory system, composed of the heart and an extensive network of blood vessels, is indispensable for maintaining life. These vessels, ranging from large arteries and veins to microscopic capillaries, ensure the delivery of oxygen, nutrients, hormones, and immune cells to every tissue in the body while simultaneously removing metabolic waste products. The formation of blood vessels, a process termed angiogenesis, is tightly regulated during embryonic development and continues, albeit at a slower rate, throughout adulthood in processes such as wound healing and tissue repair. Dysregulation of angiogenesis, on the other hand, is implicated in a wide range of pathological conditions, including cancer, cardiovascular disease, and chronic inflammatory disorders.
The understanding of blood vessel biology has significantly advanced over the past few decades, revealing the intricate molecular mechanisms and signaling pathways that govern angiogenesis. This knowledge has paved the way for the development of novel therapeutic strategies targeting angiogenesis, both to promote vessel growth in ischemic tissues (therapeutic angiogenesis) and to inhibit vessel growth in tumors (anti-angiogenesis). A particularly promising area of research involves the use of engineered blood vessel cells (R-VECs) to enhance the vascularization of transplanted tissues and organs, with the goal of improving their survival and function. In the context of islet transplantation, inadequate blood supply is a major limitation, hindering the long-term survival and function of the transplanted islets. The use of R-VECs offers a potential solution to this problem by promoting rapid and robust vascularization of the transplanted tissue.
This report aims to provide a comprehensive overview of blood vessel biology, highlighting recent advances in our understanding of angiogenesis and its therapeutic implications. We will discuss the molecular mechanisms governing vessel formation, the role of the microenvironment, and the challenges and opportunities associated with engineering functional vascular networks. We will also explore the potential of R-VECs and other strategies to improve the vascularization of transplanted tissues and address other regenerative medicine applications.
Many thanks to our sponsor Esdebe who helped us prepare this research report.
2. Developmental Angiogenesis: From Vasculogenesis to Vessel Maturation
The development of the vascular system is a complex and tightly regulated process that begins early in embryogenesis. The initial formation of blood vessels, known as vasculogenesis, involves the differentiation of mesodermal progenitor cells into angioblasts, which then coalesce to form primitive vascular networks. These networks undergo extensive remodeling and maturation, giving rise to the hierarchical structure of the mature vascular system, comprising arteries, veins, and capillaries.
2.1. Vasculogenesis and Angiogenesis: Distinct but Intertwined Processes
While vasculogenesis is the de novo formation of blood vessels from angioblasts, angiogenesis is the formation of new vessels from pre-existing ones. During embryonic development, vasculogenesis predominates in the early stages, while angiogenesis plays a crucial role in expanding and refining the initial vascular networks. In adults, angiogenesis is the primary mechanism for blood vessel formation, occurring in response to various stimuli, such as hypoxia, inflammation, and tissue injury.
2.2. Key Molecular Players in Angiogenesis
Several signaling pathways and growth factors play critical roles in regulating angiogenesis. Among the most well-studied is the vascular endothelial growth factor (VEGF) pathway. VEGF-A, the most potent angiogenic factor, binds to VEGF receptors (VEGFRs) on endothelial cells, triggering a cascade of intracellular signaling events that promote endothelial cell proliferation, migration, and survival. Other important angiogenic factors include angiopoietins (Ang-1 and Ang-2), which regulate vessel maturation and stability, and platelet-derived growth factor (PDGF), which recruits pericytes to stabilize newly formed vessels.
The Notch signaling pathway is another crucial regulator of angiogenesis, influencing endothelial cell fate and differentiation. Notch signaling controls the balance between tip cells, which lead the sprouting of new vessels, and stalk cells, which proliferate and elongate the vessel. Proper regulation of Notch signaling is essential for the formation of functional and organized vascular networks.
2.3. Vessel Maturation and Stabilization
The formation of new blood vessels is not simply a matter of endothelial cell proliferation and migration. The newly formed vessels must also undergo maturation and stabilization to become functional and long-lasting. This process involves the recruitment of pericytes, which wrap around the endothelial cells and provide structural support. Pericytes also secrete factors that promote endothelial cell survival and inhibit excessive vessel sprouting.
The extracellular matrix (ECM) also plays a crucial role in vessel maturation and stabilization. The ECM provides a scaffold for endothelial cells and pericytes and regulates their interactions. The ECM also contains growth factors and other signaling molecules that influence angiogenesis.
Many thanks to our sponsor Esdebe who helped us prepare this research report.
3. Mature Blood Vessel Function and Dysfunction
Once formed, mature blood vessels perform a wide range of essential functions, including the delivery of oxygen and nutrients, the removal of waste products, and the regulation of blood pressure. The endothelium, the single layer of cells lining the inner surface of blood vessels, plays a crucial role in regulating these functions. Endothelial cells are not merely a passive barrier but are highly active cells that secrete a variety of signaling molecules that influence vascular tone, permeability, and coagulation.
3.1. Endothelial Cell Heterogeneity
Endothelial cells are not a homogeneous population but exhibit significant heterogeneity depending on their location and function. For example, endothelial cells in the brain form a tight barrier, known as the blood-brain barrier (BBB), which restricts the passage of molecules into the brain. Endothelial cells in the liver, on the other hand, are more permeable, allowing for the exchange of nutrients and waste products between the blood and the liver tissue. This heterogeneity is essential for the proper functioning of different organs and tissues.
3.2. Endothelial Dysfunction
Endothelial dysfunction, characterized by impaired endothelial cell function, is a hallmark of many cardiovascular diseases, including atherosclerosis, hypertension, and diabetes. Endothelial dysfunction can lead to increased vascular permeability, inflammation, and thrombosis, contributing to the development and progression of these diseases. Factors that contribute to endothelial dysfunction include oxidative stress, inflammation, and dyslipidemia.
3.3. The Role of Blood Vessels in Inflammation and Immunity
Blood vessels play a crucial role in inflammation and immunity. During inflammation, endothelial cells become activated and express adhesion molecules that recruit immune cells to the site of inflammation. Endothelial cells also secrete cytokines and chemokines that further amplify the inflammatory response. In addition, blood vessels are the primary route for immune cells to enter and exit tissues during an immune response.
Many thanks to our sponsor Esdebe who helped us prepare this research report.
4. Therapeutic Angiogenesis: Promoting Blood Vessel Growth for Tissue Repair
Therapeutic angiogenesis aims to stimulate the growth of new blood vessels in ischemic tissues to improve blood supply and promote tissue repair. This approach has shown promise in treating various conditions, including peripheral artery disease, myocardial ischemia, and wound healing.
4.1. Growth Factor Delivery Strategies
One of the most common strategies for therapeutic angiogenesis is the delivery of angiogenic growth factors, such as VEGF, to the ischemic tissue. This can be achieved through various methods, including gene therapy, protein delivery, and cell-based therapies. Gene therapy involves delivering genes encoding angiogenic growth factors to the ischemic tissue, causing the cells to produce the growth factors locally. Protein delivery involves directly injecting angiogenic growth factors into the tissue. Cell-based therapies involve transplanting cells that secrete angiogenic growth factors into the tissue.
4.2. Biomaterials for Angiogenesis
Biomaterials can be used to deliver angiogenic growth factors in a controlled and sustained manner. Biomaterials can also provide a scaffold for endothelial cell attachment and migration, promoting vessel formation. Various types of biomaterials have been used for therapeutic angiogenesis, including hydrogels, scaffolds, and nanoparticles.
4.3. Challenges and Limitations of Therapeutic Angiogenesis
Despite its potential, therapeutic angiogenesis faces several challenges and limitations. One major challenge is achieving sustained and localized delivery of angiogenic growth factors. Another challenge is ensuring that the newly formed vessels are functional and stable. In some cases, therapeutic angiogenesis can lead to the formation of leaky and disorganized vessels, which can worsen the ischemia. Furthermore, the long-term safety of therapeutic angiogenesis needs to be carefully evaluated.
Many thanks to our sponsor Esdebe who helped us prepare this research report.
5. Anti-Angiogenesis: Inhibiting Blood Vessel Growth in Tumors
Anti-angiogenesis aims to inhibit the growth of new blood vessels in tumors to starve them of oxygen and nutrients, thereby suppressing tumor growth and metastasis. This approach has become an important strategy in cancer therapy, and several anti-angiogenic drugs have been approved for clinical use.
5.1. VEGF Inhibitors
The most common anti-angiogenic drugs are VEGF inhibitors, which block the binding of VEGF to its receptors on endothelial cells. These drugs include monoclonal antibodies that target VEGF, such as bevacizumab, and small-molecule inhibitors that target VEGFR tyrosine kinases, such as sunitinib and sorafenib. VEGF inhibitors have shown efficacy in treating various types of cancer, including colorectal cancer, lung cancer, and kidney cancer.
5.2. Other Anti-Angiogenic Strategies
Besides VEGF inhibitors, other anti-angiogenic strategies are being developed, including inhibitors of other angiogenic factors, such as angiopoietins and PDGF, and inhibitors of endothelial cell signaling pathways, such as the Notch pathway. These strategies aim to target different aspects of angiogenesis and may be effective in tumors that are resistant to VEGF inhibitors.
5.3. Challenges and Limitations of Anti-Angiogenesis
Anti-angiogenesis also faces several challenges and limitations. One major challenge is the development of resistance to anti-angiogenic drugs. Tumors can develop resistance by upregulating other angiogenic factors or by recruiting alternative blood supply pathways. Another challenge is the potential for anti-angiogenic drugs to promote tumor invasion and metastasis in some cases. Furthermore, anti-angiogenic drugs can have significant side effects, including hypertension, bleeding, and wound healing problems.
Many thanks to our sponsor Esdebe who helped us prepare this research report.
6. Engineered Blood Vessel Cells (R-VECs): A Promising Tool for Regenerative Medicine
Engineered blood vessel cells (R-VECs) are cells that have been genetically modified or otherwise manipulated to enhance their angiogenic properties. R-VECs can be derived from various cell sources, including endothelial progenitor cells (EPCs), mesenchymal stem cells (MSCs), and induced pluripotent stem cells (iPSCs). R-VECs can be used to promote vascularization of transplanted tissues and organs, enhance wound healing, and treat other conditions characterized by impaired blood supply.
6.1. Generation and Characterization of R-VECs
R-VECs can be generated using various techniques, including viral transduction, non-viral gene transfer, and CRISPR-Cas9 gene editing. The angiogenic properties of R-VECs can be enhanced by overexpressing angiogenic growth factors, such as VEGF, or by silencing genes that inhibit angiogenesis. R-VECs are typically characterized by their expression of endothelial cell markers, their ability to form blood vessels in vitro and in vivo, and their secretion of angiogenic growth factors.
6.2. Application of R-VECs in Islet Transplantation
In the context of islet transplantation, R-VECs can be used to promote rapid and robust vascularization of the transplanted islets, improving their survival and function. R-VECs can be co-transplanted with the islets or delivered separately to the transplantation site. Several studies have shown that R-VECs can significantly improve the outcome of islet transplantation in animal models.
6.3. Potential and Challenges of R-VEC Technology
R-VEC technology holds great promise for regenerative medicine, but several challenges need to be addressed before it can be widely applied in the clinic. One major challenge is ensuring the safety and efficacy of R-VECs. The long-term effects of R-VECs on the host tissue need to be carefully evaluated. Another challenge is the scalability of R-VEC production. Large numbers of R-VECs are needed for clinical applications, and efficient and cost-effective methods for R-VEC production need to be developed.
Many thanks to our sponsor Esdebe who helped us prepare this research report.
7. Microfabrication Techniques for Creating Vascular Networks
Microfabrication techniques, such as microfluidics and 3D bioprinting, offer new possibilities for creating complex and functional vascular networks in vitro and in vivo. These techniques allow for precise control over the architecture and composition of the vascular networks, enabling the creation of customized vascular grafts and tissue-engineered constructs.
7.1. Microfluidic Devices for Angiogenesis Research
Microfluidic devices can be used to study angiogenesis in a controlled and reproducible manner. These devices allow for the creation of microenvironments that mimic the in vivo conditions and enable the investigation of the effects of various factors on angiogenesis, such as growth factors, chemokines, and mechanical forces.
7.2. 3D Bioprinting of Vascularized Tissues
3D bioprinting allows for the creation of complex, three-dimensional tissues with integrated vascular networks. This technology involves the layer-by-layer deposition of cells, biomaterials, and growth factors to create functional tissue constructs. 3D bioprinting has shown promise in creating vascularized skin grafts, bone grafts, and other tissue-engineered constructs.
7.3. Challenges and Future Directions of Microfabrication
Microfabrication techniques for creating vascular networks are still in their early stages of development, and several challenges need to be addressed. One major challenge is creating vascular networks that are functional and long-lasting. Another challenge is ensuring that the microfabricated tissues are biocompatible and integrate properly with the host tissue. Future research will focus on developing more sophisticated microfabrication techniques and on optimizing the design and composition of vascularized tissues.
Many thanks to our sponsor Esdebe who helped us prepare this research report.
8. Conclusion and Future Perspectives
Blood vessels are essential for tissue homeostasis and repair, and understanding their biology is crucial for developing new therapeutic strategies for a wide range of diseases. Significant progress has been made in recent years in our understanding of angiogenesis and its therapeutic applications. Therapeutic angiogenesis holds promise for treating ischemic diseases, while anti-angiogenesis has become an important strategy in cancer therapy. The use of engineered blood vessel cells (R-VECs) and microfabrication techniques offers new possibilities for creating functional vascular networks and for improving the outcome of transplantation and other regenerative medicine applications.
Despite these advances, several challenges remain. Achieving sustained and localized delivery of angiogenic growth factors, ensuring the stability and functionality of newly formed vessels, and overcoming resistance to anti-angiogenic drugs are major challenges that need to be addressed. Future research will focus on developing more targeted and effective therapies that promote or inhibit angiogenesis in a controlled and predictable manner. The integration of advanced technologies, such as gene editing, biomaterials, and microfabrication, will pave the way for the development of personalized and regenerative medicine approaches that harness the power of blood vessels to treat a wide range of diseases.
Many thanks to our sponsor Esdebe who helped us prepare this research report.
References
- Carmeliet, P. (2003). Angiogenesis in health and disease. Nature Medicine, 9(6), 653-660.
- Folkman, J. (1971). Tumor angiogenesis: therapeutic implications. New England Journal of Medicine, 285(21), 1182-1186.
- Augustin, H. G., & Koh, G. Y. (2017). Organotypic angiogenesis: beyond endothelial cell sprouting. Science, 357(6358), 1324-1329.
- Potente, M., Gerhardt, H., & Carmeliet, P. (2011). Basic and therapeutic aspects of angiogenesis. Cell, 146(6), 873-887.
- Risau, W. (1997). Mechanisms of angiogenesis. Nature, 386(6626), 671-674.
- Simons, M. (2005). Angiogenesis: where do we stand today?. Circulation, 111(12), 1556-1566.
- Carmeliet, P., & Jain, R. K. (2011). Molecular mechanisms and clinical applications of angiogenesis. Nature, 473(7347), 298-307.
- Ribatti, D., Nico, B., & Crivellato, E. (2015). The history of angiogenesis research. Angiogenesis, 18(1), 1-9.
- Quertermous, T. (2003). Therapeutic angiogenesis: an unfulfilled promise. Journal of the American College of Cardiology, 41(10), 1663-1665.
- Jain, R. K. (2005). Normalization of tumor vasculature: an emerging concept in antiangiogenic therapy. Science, 307(5706), 58-62.
- D’Amore, P. A. (2007). Mechanisms of retinal and choroidal angiogenesis. Investigative Ophthalmology & Visual Science, 48(5), 2323-2330.
- Carmeliet, P. (2005). VEGF as a key mediator of angiogenesis in cancer. Oncology, 69 Suppl 3, 4-10.
- Ferrara, N., Gerber, H. P., & LeCouter, J. (2003). The biology of VEGF and its receptors. Nature Medicine, 9(6), 669-676.
- Yancopoulos, G. D., Davis, S., Gale, N. W., Rudge, J. S., Wiegand, S. J., & Holash, J. (2000). Vascular-specific growth factors and angiogenesis. Nature, 407(6804), 242-248.
- Adams, R. H., & Alitalo, K. (2007). Molecular regulation of angiogenesis and lymphangiogenesis. Nature Reviews Molecular Cell Biology, 8(6), 464-478.
- Cleaver, O., & Melton, D. A. (2003). Endothelial signaling in vertebrate development. Nature Cell Biology, 5(8), 661-666.
- Eelen, G., de Zeeuw, P., Simons, M., & Carmeliet, P. (2015). Endothelial cell metabolism in normal and diseased vasculature. Circulation Research, 116(7), 1231-1244.
- Aird, W. C. (2007). Endothelial cell heterogeneity. Cold Spring Harbor Perspectives in Biology, 4(1), a006429.
- Deanfield, J. E., Halcox, J. P., & Rabelink, T. J. (2007). Endothelial function and dysfunction: testing and clinical relevance. Circulation, 115(9), 1285-1295.
- Libby, P., Ridker, P. M., & Hansson, G. K. (2011). Inflammation in atherosclerosis. Nature, 473(7347), 317-325.
- Carmeliet, P., & De Smet, F. (2011). Revascularization therapy for ischemic diseases: a critical appraisal of pros and cons. Trends in Cardiovascular Medicine, 21(2), 41-46.
- Ferrara, N. (2004). Vascular endothelial growth factor: basic science and clinical progress. Endocrine Reviews, 25(4), 581-611.
- Jain, R. K. (2001). Delivery of molecular and cellular medicine to solid tumors. Science, 296(5567), 321-324.
- Folkman, J. (2002). Angiogenesis: an overview. Seminars in Oncology, 29(6 Suppl 16), 6-15.
- Bergers, G., & Benjamin, L. E. (2003). Tumor microenvironment: the role of angiogenesis in cancer progression. Nature Reviews Cancer, 3(6), 401-412.
- Kerbel, R. S. (2008). Antiangiogenic therapy: a universal chemosensitization strategy for cancer?. Science, 307(5709), 417-423.
- Ellis, L. M., & Hicklin, D. J. (2008). VEGF-targeted therapy: mechanisms of anti-tumour activity. Nature Reviews Cancer, 8(8), 579-591.
- Carmeliet, P., & Jain, R. K. (2000). Angiogenesis in cancer and other diseases. Nature, 407(6801), 249-257.
- Tepper, O. M., Unger, R. E., & Nerem, R. M. (2013). Directed angiogenesis in tissue engineering. Trends in Biotechnology, 31(12), 705-713.
- Laschke, M. W., & Menger, M. D. (2016). In vivo assessment of microvascular response to biomaterials. Biomaterials, 80, 1-15.
- Murphy, S. V., & Atala, A. (2014). 3D bioprinting of tissues and organs. Nature Biotechnology, 32(8), 773-785.
- Nguyen, D. T., & Bhatia, S. N. (2015). Three-dimensional microtissue arrays for cell-based assays. Nature Protocols, 10(1), 1-13.
This report highlights the exciting potential of R-VECs in islet transplantation. Considering the challenges of scalability in R-VEC production for clinical applications, what are the latest advancements in bioreactor technology or cell culture methods to address this critical need?
That’s a great point! Scalability is indeed a key hurdle. There’s exciting progress in optimizing 3D culture systems and microcarrier technology to increase R-VEC yields. Improved bioreactor designs with better control over oxygen and nutrient gradients are also showing promise for large-scale production. This will accelerate clinical translation.
Editor: MedTechNews.Uk
Thank you to our Sponsor Esdebe
The insights into the molecular mechanisms of angiogenesis are fascinating. How might a deeper understanding of endothelial cell metabolism, particularly in diseased vasculature, inform more targeted therapeutic strategies for promoting vessel normalization?