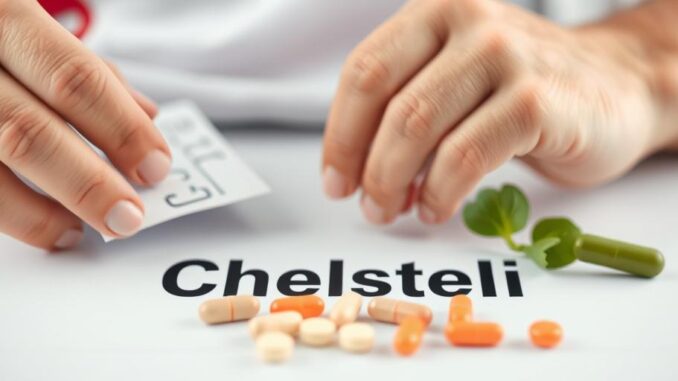
Abstract
Cholesterol, an essential structural component of cell membranes and a precursor for steroid hormones and bile acids, plays a pivotal role in maintaining cellular function and overall metabolic health. Dysregulation of cholesterol homeostasis, primarily manifested as elevated low-density lipoprotein cholesterol (LDL-C), is a major risk factor for cardiovascular disease (CVD). This review provides a comprehensive overview of cholesterol metabolism, encompassing biosynthesis, uptake, efflux, and transport mechanisms. We delve into the intricate regulatory pathways governing cholesterol levels, highlighting the roles of key transcription factors, enzymes, and signaling molecules. Furthermore, we explore the profound influence of maternal cholesterol levels during pregnancy on fetal development and offspring cardiovascular health. Finally, we discuss current and emerging therapeutic strategies targeting cholesterol metabolism, with a focus on personalized approaches to effectively manage dyslipidemia and mitigate CVD risk.
Many thanks to our sponsor Esdebe who helped us prepare this research report.
1. Introduction
Cholesterol, a sterol lipid, is indispensable for mammalian life. Beyond its structural role in cell membranes, modulating fluidity and permeability, cholesterol serves as a precursor for the synthesis of vital biomolecules, including steroid hormones (e.g., cortisol, aldosterone, testosterone, estrogen) and bile acids, which are crucial for lipid digestion and absorption [1]. Maintaining cholesterol homeostasis, a delicate balance between synthesis, uptake, and excretion, is therefore paramount for optimal physiological function. Dysregulation of this balance, particularly elevated levels of LDL-C, is a well-established and modifiable risk factor for atherosclerotic CVD, including coronary artery disease, stroke, and peripheral artery disease [2].
While genetic factors significantly influence an individual’s predisposition to dyslipidemia, environmental factors, such as diet and lifestyle, play a crucial modulatory role. The complex interplay between genetic and environmental influences necessitates a multifaceted approach to cholesterol management. This review aims to provide a detailed examination of cholesterol metabolism, its regulation, and the maternal influence on offspring cholesterol levels, concluding with a discussion of existing and emerging therapeutic strategies. A key focus will be on the sophisticated regulatory mechanisms governing cholesterol homeostasis, considering both cellular and systemic levels.
Many thanks to our sponsor Esdebe who helped us prepare this research report.
2. Cholesterol Metabolism: A Dynamic Equilibrium
Cholesterol metabolism is a complex, multi-faceted process involving synthesis, uptake, transport, and excretion. Understanding each of these components is critical for comprehending the mechanisms underlying cholesterol dysregulation.
2.1. Cholesterol Biosynthesis
The de novo synthesis of cholesterol occurs primarily in the liver and, to a lesser extent, in other tissues. The process involves a series of enzymatic reactions starting with acetyl-CoA and culminating in the formation of cholesterol [3]. The rate-limiting step in cholesterol biosynthesis is catalyzed by 3-hydroxy-3-methylglutaryl-CoA reductase (HMGCR), which converts HMG-CoA to mevalonate. HMGCR activity is tightly regulated by a variety of factors, including intracellular cholesterol levels, hormones (e.g., insulin, glucagon), and dietary cholesterol intake [4].
The sterol regulatory element-binding protein 2 (SREBP-2) is a key transcription factor that regulates the expression of HMGCR and other genes involved in cholesterol synthesis. When intracellular cholesterol levels are low, SREBP-2 is cleaved and translocates to the nucleus, where it binds to sterol regulatory elements (SREs) in the promoter regions of target genes, thereby increasing their transcription [5]. Conversely, high intracellular cholesterol levels suppress SREBP-2 processing and activation, leading to decreased expression of cholesterol biosynthetic genes.
2.2. Cholesterol Uptake
Cells acquire cholesterol from two primary sources: de novo synthesis and uptake from the extracellular environment. LDL-C, the major carrier of cholesterol in the circulation, is taken up by cells via the LDL receptor (LDLR) [6]. The LDLR binds to apolipoprotein B-100 (apoB-100) on the surface of LDL particles, initiating receptor-mediated endocytosis. The resulting endosome fuses with a lysosome, where LDL-C is hydrolyzed, releasing free cholesterol into the cytoplasm.
Similar to HMGCR, the expression of the LDLR is also regulated by SREBP-2. In addition, proprotein convertase subtilisin/kexin type 9 (PCSK9) plays a crucial role in regulating LDLR levels. PCSK9 binds to the LDLR on the cell surface, promoting its degradation in the lysosome, thereby reducing the number of LDLRs available for LDL-C uptake [7]. This process is a significant target for therapeutic intervention.
2.3. Cholesterol Efflux
Cholesterol efflux, the process by which cells remove excess cholesterol, is critical for preventing intracellular cholesterol accumulation and maintaining cellular homeostasis. ATP-binding cassette transporter A1 (ABCA1) is a key protein involved in cholesterol efflux. ABCA1 mediates the transfer of cholesterol and phospholipids from the cell membrane to apolipoprotein A-I (apoA-I), the major protein component of high-density lipoprotein (HDL) [8]. This process initiates the formation of nascent HDL particles, which can then acquire more cholesterol from other cells and tissues.
Another important transporter involved in cholesterol efflux is ATP-binding cassette transporter G1 (ABCG1), which mediates the efflux of cholesterol to mature HDL particles [9]. The liver X receptor (LXR), a nuclear receptor activated by oxysterols (oxidized cholesterol derivatives), regulates the expression of both ABCA1 and ABCG1, providing a feedback mechanism to enhance cholesterol efflux when intracellular cholesterol levels are high [10].
2.4. Cholesterol Transport
Cholesterol is transported throughout the body via lipoproteins, complex particles composed of lipids and proteins. The major lipoproteins include chylomicrons, very-low-density lipoprotein (VLDL), LDL, and HDL [11]. Chylomicrons transport dietary triglycerides and cholesterol from the intestine to the liver and other tissues. VLDL, synthesized in the liver, transports triglycerides to peripheral tissues. As VLDL particles lose triglycerides, they are converted to LDL. LDL-C delivers cholesterol to cells throughout the body.
HDL plays a crucial role in reverse cholesterol transport, the process by which excess cholesterol is removed from peripheral tissues and transported back to the liver for excretion in bile [12]. HDL particles acquire cholesterol from cells via ABCA1 and ABCG1, as described above. The enzyme lecithin-cholesterol acyltransferase (LCAT) esterifies free cholesterol on HDL particles, forming cholesteryl esters that are sequestered in the core of the particle, allowing HDL to acquire more cholesterol. HDL-C can then deliver cholesterol to the liver directly or indirectly, via transfer to other lipoproteins. The current view suggests more nuance as the function of HDL is more complex than simply acting as a cholesterol shuttle. The impact on RCT depends on factors like HDL particle size, composition, and functionality which can be impaired in certain disease states [13].
Many thanks to our sponsor Esdebe who helped us prepare this research report.
3. Regulatory Mechanisms of Cholesterol Homeostasis
Maintaining cholesterol homeostasis requires a complex interplay of regulatory mechanisms operating at both the cellular and systemic levels. These mechanisms involve transcriptional control, enzymatic regulation, hormonal signaling, and feedback loops.
3.1. Transcriptional Regulation
As mentioned earlier, SREBP-2 plays a central role in regulating the expression of genes involved in cholesterol synthesis and uptake [5]. However, other transcription factors also contribute to cholesterol homeostasis. For example, the LXRs regulate the expression of genes involved in cholesterol efflux and bile acid synthesis [10]. The peroxisome proliferator-activated receptors (PPARs) also influence cholesterol metabolism by regulating the expression of genes involved in lipid transport and oxidation [14].
Furthermore, microRNAs (miRNAs), small non-coding RNA molecules, can regulate gene expression at the post-transcriptional level. Several miRNAs have been shown to target genes involved in cholesterol metabolism, including HMGCR, LDLR, ABCA1, and ABCG1 [15]. These miRNAs can either increase or decrease the expression of their target genes, thereby influencing cholesterol levels.
3.2. Enzymatic Regulation
The activity of several key enzymes involved in cholesterol metabolism is regulated by a variety of factors. HMGCR activity is regulated by phosphorylation, with phosphorylation inhibiting its activity [4]. AMP-activated protein kinase (AMPK), a cellular energy sensor, phosphorylates and inactivates HMGCR when cellular energy levels are low. Insulin stimulates HMGCR activity by promoting its dephosphorylation. Glucagon has the opposite effect.
LCAT activity is regulated by the composition of HDL particles [16]. HDL particles enriched in apoA-I are more effective at activating LCAT. Cholesteryl ester transfer protein (CETP) mediates the transfer of cholesteryl esters from HDL to VLDL and LDL in exchange for triglycerides. CETP activity is influenced by plasma lipid levels and can impact HDL-C levels. The clinical utility of CETP inhibitors has been complex, with mixed results regarding cardiovascular outcomes [17].
3.3. Hormonal Signaling
Several hormones play a role in regulating cholesterol metabolism. Insulin stimulates cholesterol synthesis and reduces LDL-C levels [18]. Thyroid hormone increases LDLR expression and lowers LDL-C levels [19]. Glucocorticoids can increase cholesterol synthesis and raise LDL-C levels [20]. Estrogens generally have a beneficial effect on cholesterol levels, increasing HDL-C and decreasing LDL-C [21].
3.4. Feedback Loops
Intracellular cholesterol levels exert a negative feedback effect on cholesterol synthesis and uptake [5]. High intracellular cholesterol levels suppress SREBP-2 processing and activation, leading to decreased expression of HMGCR and LDLR. Conversely, low intracellular cholesterol levels stimulate SREBP-2 activation, increasing the expression of these genes. This feedback loop helps to maintain cholesterol homeostasis by ensuring that cells have adequate cholesterol levels without accumulating excess cholesterol.
Many thanks to our sponsor Esdebe who helped us prepare this research report.
4. Maternal Influence on Offspring Cholesterol Levels and Cardiovascular Health
The maternal environment during pregnancy has a profound impact on fetal development and long-term health outcomes. Maternal cholesterol levels, in particular, can significantly influence fetal cholesterol metabolism and offspring cardiovascular health [22].
4.1. Fetal Cholesterol Metabolism
The fetus relies on maternal cholesterol for its development, particularly during the first trimester when fetal cholesterol synthesis is relatively low [23]. Cholesterol is transported across the placenta via lipoprotein particles, primarily LDL. Maternal hypercholesterolemia can lead to increased cholesterol transfer to the fetus, potentially affecting fetal cholesterol metabolism and cardiovascular development. Studies have shown that elevated maternal cholesterol levels during pregnancy are associated with increased cord blood cholesterol levels in newborns [24].
4.2. Programming Effects
Maternal hypercholesterolemia during pregnancy can induce epigenetic modifications in the fetal genome, leading to altered gene expression patterns that persist into adulthood [25]. These epigenetic changes can affect genes involved in cholesterol metabolism, inflammation, and vascular function, increasing the offspring’s susceptibility to cardiovascular disease later in life. This phenomenon, known as developmental programming, highlights the importance of maternal metabolic health during pregnancy.
Animal studies have demonstrated that maternal high-fat diets during pregnancy can lead to increased cholesterol levels and atherosclerotic lesions in the offspring [26]. Human studies have also shown that children born to mothers with hypercholesterolemia are at increased risk of developing dyslipidemia and cardiovascular disease in adulthood [27].
4.3. Mitigation Strategies
Managing maternal cholesterol levels during pregnancy is crucial for optimizing fetal development and offspring cardiovascular health. Lifestyle modifications, such as adopting a healthy diet and engaging in regular physical activity, can help to lower cholesterol levels [28]. However, the use of cholesterol-lowering medications, such as statins, during pregnancy is generally contraindicated due to potential teratogenic effects [29].
Emerging research suggests that interventions targeting maternal gut microbiota composition may be a promising strategy for improving maternal and offspring metabolic health [30]. Probiotics and prebiotics have been shown to modulate gut microbiota composition and improve cholesterol metabolism in both animal and human studies. However, further research is needed to determine the optimal strategies for modulating maternal gut microbiota during pregnancy to improve offspring cardiovascular health.
Many thanks to our sponsor Esdebe who helped us prepare this research report.
5. Therapeutic Strategies for Managing Cholesterol
The primary goal of cholesterol-lowering therapy is to reduce the risk of atherosclerotic CVD. Several therapeutic strategies are available, including lifestyle modifications and pharmacological interventions.
5.1. Lifestyle Modifications
Lifestyle modifications are the cornerstone of cholesterol management. A healthy diet, regular physical activity, weight management, and smoking cessation can all significantly lower LDL-C levels and reduce CVD risk [28]. A diet rich in fruits, vegetables, whole grains, and lean protein, and low in saturated and trans fats, can lower LDL-C levels by 10-20% [31]. Regular physical activity can increase HDL-C levels and lower LDL-C levels [32].
5.2. Pharmacological Interventions
Statins are the most widely prescribed cholesterol-lowering medications. Statins inhibit HMGCR, the rate-limiting enzyme in cholesterol synthesis, leading to decreased intracellular cholesterol levels and increased LDLR expression [33]. This results in a reduction in LDL-C levels and a decreased risk of CVD. Statins have been shown to reduce the risk of heart attack, stroke, and death in both primary and secondary prevention trials [34].
Ezetimibe is a cholesterol absorption inhibitor that blocks the absorption of cholesterol from the intestine [35]. Ezetimibe can be used alone or in combination with a statin to further lower LDL-C levels. PCSK9 inhibitors are a newer class of cholesterol-lowering medications that inhibit PCSK9, a protein that degrades the LDLR [7]. PCSK9 inhibitors significantly lower LDL-C levels and have been shown to reduce the risk of CVD events [36].
Bile acid sequestrants bind to bile acids in the intestine, preventing their reabsorption and promoting their excretion [37]. This increases the demand for cholesterol to synthesize new bile acids, leading to decreased LDL-C levels. Fibrates activate PPARα, a nuclear receptor that regulates lipid metabolism [14]. Fibrates primarily lower triglyceride levels and increase HDL-C levels. Niacin (nicotinic acid) reduces VLDL synthesis, leading to decreased LDL-C and triglyceride levels, and increased HDL-C levels [38]. However, niacin is associated with significant side effects, including flushing and liver toxicity.
5.3. Emerging Therapies
Several emerging therapies are being developed to target cholesterol metabolism. Inclisiran is a small interfering RNA (siRNA) that inhibits PCSK9 synthesis in the liver [39]. Inclisiran is administered as a subcutaneous injection twice a year and has been shown to effectively lower LDL-C levels. Bempedoic acid is an ATP citrate lyase (ACL) inhibitor that inhibits cholesterol synthesis upstream of HMGCR [40]. Bempedoic acid can be used alone or in combination with a statin to lower LDL-C levels. Anacetrapib, a CETP inhibitor, was shown to significantly raise HDL levels, but its clinical development was terminated due to concerns about off-target effects [41]. Research into therapies targeting lipoprotein(a) [Lp(a)], a genetically determined risk factor for CVD, is ongoing. Oligonucleotide therapies are showing promise in lowering Lp(a) levels [42].
5.4. Personalized Approaches
The management of cholesterol is becoming increasingly personalized. Genetic testing can identify individuals at high risk of developing dyslipidemia and CVD [43]. This information can be used to tailor treatment strategies to individual needs. For example, individuals with genetic variants that increase PCSK9 expression may benefit more from PCSK9 inhibitor therapy. Furthermore, the gut microbiome’s role in cholesterol metabolism is being actively investigated, potentially opening the door to personalized dietary and probiotic interventions [44].
Many thanks to our sponsor Esdebe who helped us prepare this research report.
6. Conclusion
Cholesterol homeostasis is a complex process involving multiple regulatory mechanisms. Dysregulation of cholesterol metabolism, particularly elevated LDL-C levels, is a major risk factor for CVD. Understanding the intricate pathways governing cholesterol levels is crucial for developing effective strategies to prevent and treat dyslipidemia. Maternal cholesterol levels during pregnancy have a profound impact on fetal development and offspring cardiovascular health, highlighting the importance of optimizing maternal metabolic health. Current therapeutic strategies for managing cholesterol include lifestyle modifications and pharmacological interventions, such as statins, ezetimibe, and PCSK9 inhibitors. Emerging therapies, such as inclisiran and bempedoic acid, offer new hope for individuals who do not achieve adequate LDL-C control with existing treatments. Personalized approaches to cholesterol management, based on genetic testing and individual risk factors, are becoming increasingly important. Future research should focus on further elucidating the complex interplay of genetic, environmental, and lifestyle factors that influence cholesterol metabolism, with the goal of developing more effective and personalized strategies to prevent and treat CVD. Targeting the gut microbiome, understanding HDL functionality in various disease states, and the development of therapies that target Lp(a) are promising avenues for future research and clinical advancements.
Many thanks to our sponsor Esdebe who helped us prepare this research report.
References
[1] Berg, J. M., Tymoczko, J. L., & Stryer, L. (2002). Biochemistry. 5th edition. New York: W H Freeman.
[2] Ference, B. A., Ginsberg, H. N., Graham, I., Ray, K. K., Packard, C. J., Bruckert, E., … & Baigent, C. (2017). Low-density lipoproteins cause atherosclerotic cardiovascular disease. 1. Evidence from genetic, epidemiologic, and clinical studies. European heart journal, 38(32), 2459-2472.
[3] Goldstein, J. L., & Brown, M. S. (1990). Regulation of the mevalonate pathway. Nature, 343(6257), 425-430.
[4] Horton, J. D., Goldstein, J. L., & Brown, M. S. (2002). SREBPs: activators of the complete program of cholesterol and fatty acid synthesis in the liver. Journal of Clinical Investigation, 109(9), 1125-1131.
[5] Brown, M. S., & Goldstein, J. L. (1997). The SREBP pathway: regulation of cholesterol metabolism by proteolysis of a membrane-bound transcription factor. Cell, 89(3), 331-340.
[6] Brown, M. S., & Goldstein, J. L. (1986). A receptor-mediated pathway for cholesterol homeostasis. Science, 232(4746), 34-47.
[7] Horton, J. D., Cohen, J. C., & Hobbs, H. H. (2007). Molecular biology of PCSK9. Circulation, 115(2), 200-207.
[8] Oram, J. F., & Vaughan, A. M. (2000). ATP-binding cassette transporter A1: a cell cholesterol transporter that protects against cardiovascular disease. Arteriosclerosis, thrombosis, and vascular biology, 20(5), 1263-1272.
[9] Wang, N., Lan, D., Gerbod-Rabot, S., Smart, A., Tall, A. R., & Glass, C. K. (2004). ATP-binding cassette transporters G1 and A1 mediate cellular cholesterol removal to high-density lipoproteins. Proceedings of the National Academy of Sciences, 101(26), 9774-9779.
[10] Peet, D. J., Janowski, B. A., & Mangelsdorf, D. J. (1998). Activation of gene expression by peroxisome proliferator-activated receptors. Proceedings of the National Academy of Sciences, 95(22), 12979-12983.
[11] Feingold, K. R. (2000). Introduction to Lipids and Lipoproteins. In Endotext [Internet]. MDText. com, Inc.
[12] Tall, A. R. (1990). Plasma high-density lipoproteins. Metabolism and relationship to atherogenesis. Journal of Clinical Investigation, 86(2), 379-384.
[13] Rohatgi, A. (2014). High-density lipoprotein cholesterol and cardiovascular disease: an evidence-based reappraisal. Circulation, 129(24), 2573-2577.
[14] Fruchart, J. C., Duriez, P., & Staels, B. (2001). Peroxisome proliferator-activated receptors and atherosclerosis. The Lancet, 357(9256), 555-559.
[15] Rotllan, N., Ramírez, C. M., Fernández-Hernando, C., & Fernández-Hernando, C. (2015). MicroRNAs in lipid metabolism. Current opinion in lipidology, 26(4), 301-309.
[16] Jonas, A. (2000). Lecithin cholesterol acyltransferase. Biochimica et Biophysica Acta (BBA)-Lipids and Lipid Metabolism, 1529(1-3), 245-256.
[17] Barter, P. J., Rye, K. A., & Nicholls, S. J. (2016). CETP inhibitors: past failures and future hopes. Current opinion in lipidology, 27(4), 341-346.
[18] Gibbons, G. F., & Ramsay, R. R. (1995). The effect of insulin on hepatic fatty acid metabolism. Biochemical Journal, 309(Pt 1), 1-9.
[19] Duntas, L. H., Brenta, G., & Thyroid, A. (2012). A Thyroid, a Lipid, and an Artery. Hormone and Metabolic Research, 44(03), 171-177.
[20] Havel, R. J. (1984). The role of the liver in hyperlipoproteinemia and hyperlipidemia. Advances in Internal Medicine, 29, 277-295.
[21] Stevenson, J. C., Crook, D., Godsland, I. F., & Collins, P. (1993). Influence of age and menopause on serum lipids and lipoproteins in healthy women. Atherosclerosis, 98(1), 83-90.
[22] Herrera, E., Ortega-Senovilla, H., & Gómez-Valdés, A. (2006). Maternal triglycerides and placental function. American Journal of Obstetrics and Gynecology, 194(3 Suppl), S20-S26.
[23] Carr, B. R., MacDonald, P. C., & Simpson, E. R. (1981). The regulation of de novo synthesis of cholesterol in the human fetal adrenal gland. Endocrinology, 108(3), 1072-1076.
[24] Gidding, S. S., Sniderman, A., Anand, S., Beaulac-Baillargeon, L., Blackett, P., D’Souza, M., … & Thanassoulis, G. (2015). Familial hypercholesterolemia: the underrecognized cause of early cardiovascular disease. Canadian Journal of Cardiology, 31(8), 900-906.
[25] Godfrey, K. M., Sheppard, A., Gluckman, P. D., & Lillycrop, K. A. (2017). Epigenetic mechanisms and the mismatch concept of health and disease. The Lancet Diabetes & Endocrinology, 5(3), 227-234.
[26] van der Heijden, O. A., Rijken, M., van Dijk, T. H., Groen, A. K., & Postma, M. S. (2011). Programming of cardiovascular risk factors: the role of maternal diet. Journal of Human Hypertension, 25(4), 219-228.
[27] Olsen, S. F., Halldorsson, T. I., Willett, W. C., Knudsen, V. K., Stripp, C., Hansen, H. S., & Grandjean, P. (2007). High blood pressure in childhood, adolescence and early adulthood is associated with grandmaternal seafood intake during pregnancy. Journal of Hypertension, 25(3), 579-586.
[28] Eckel, R. H., Jakicic, J. M., Ard, J. D., de Jesus, J. M., Houston Miller, N., Hubbard, V. S., … & Yanovski, S. Z. (2014). 2013 AHA/ACC guideline on lifestyle management to reduce cardiovascular risk: a report of the American College of Cardiology/American Heart Association Task Force on Practice Guidelines. Circulation, 129(25 Suppl 2), S76-S99.
[29] Newman, T. B., & Hulley, S. B. (1996). Carcinogenicity of lipid-lowering drugs. JAMA, 275(1), 55-60.
[30] Gomez Arango, L. F., Barrett, H. L., Callaway, L. K., Hort, W. E., & Morrison, M. (2016). Gut microbiota in pregnancy and its relationship to gestational diabetes mellitus. Journal of Reproductive Immunology, 116, 44-52.
[31] Anderson, J. W., Bush, H. M., Thornton, J. C., Porter, G. E., & Keyserlingk, J. R. (1992). Dietary fiber is effective in treatment of hypercholesterolemia. The American Journal of Clinical Nutrition, 55(1), 49-63.
[32] Kelley, G. A., & Kelley, K. S. (2008). Exercise and lipids: a critical review of the clinical literature. Journal of the American College of Nutrition, 27(1), 1-32.
[33] Istvan, E. S. (2002). Mechanism of action of statins. Circulation, 105(22), 2665-2672.
[34] Baigent, C., Blackwell, L., Emberson, J., Holland, L. E., Reith, C., Bhala, N., … & Collins, R. (2010). Efficacy and safety of more intensive lowering of LDL cholesterol: a meta-analysis of data from 170 000 participants in 26 randomised trials. The Lancet, 376(9753), 1670-1681.
[35] Davis, H. R., Zhu, L. J., Hoos, L. M., Tetzloff, G., Maguire, M., Kozarich, J. W., & Marucci, J. D. (2001). Ezetimibe, a novel cholesterol absorption inhibitor, reduces plasma cholesterol in rats and monkeys. Atherosclerosis, 158(2), 467-476.
[36] Sabatine, M. S., Giugliano, R. P., Keech, A. C., Honarpour, N., Wiviott, S. D., Murphy, S. A., … & Sever, P. S. (2017). Evolocumab and clinical outcomes in patients with cardiovascular disease. New England Journal of Medicine, 376(18), 1713-1722.
[37] Toth, P. P. (2016). Bile acid sequestrants and their role in managing dyslipidemia. Expert opinion on drug safety, 15(3), 377-391.
[38] Goldberg, A. C., Jacobson, T. A., & Safar, N. M. (2010). Niacin in patients with dyslipidemia: new perspectives on mechanism of action and safety. Mayo Clinic Proceedings, 85(6), 553-561.
[39] Ray, K. K., Landmesser, U., Leiter, L. A., Kallend, D., Dufour, R., Earthy, D., … & Wright, R. S. (2017). Inclisiran in patients at high cardiovascular risk with elevated LDL cholesterol. New England Journal of Medicine, 376(15), 1430-1440.
[40] Pinkosky, S. L., Newton, R. S., Day, E. A., Ford, R. J., Lhotak, S., Monsalvo, J. L., … & Zambrowicz, B. P. (2013). Liver-specific ATP-citrate lyase inhibition lowers plasma cholesterol and triglycerides in hypercholesterolemic mice and dogs. American Journal of Physiology-Gastrointestinal and Liver Physiology, 304(10), G1309-G1316.
[41] HPS3/TIMI55–REVEAL Collaborative Group. (2017). Effects of anacetrapib in patients with atherosclerotic vascular disease. New England Journal of Medicine, 377(13), 1217-1227.
[42] Viney, N. J., van Capelleveen, J. C., Geary, R. S., Xia, S., Tsimikas, S., Witztum, J. L., … & Hughes, S. G. (2016). Antisense oligonucleotides targeting apolipoprotein (a) in people with raised lipoprotein (a): two randomised, double-blind, placebo-controlled, dose-ranging trials. The Lancet, 388(10057), 2239-2249.
[43] Talmud, P. J. (2004). Genetic determinants of plasma lipid levels. Clinica Chimica Acta, 343(1-2), 1-16.
[44] Spencer, M., & Vitetta, L. (2017). Gut microbiota: implications for human health. Australian family physician, 46(9), 646.
Fascinating deep dive into cholesterol! I’m suddenly wondering, with all this talk of personalized approaches, when we’ll see at-home cholesterol “hacking” kits. Imagine brewing your own bile acid sequestrants in the kitchen. Side effects may include unexpected enlightenment.
That’s a fantastic point! The idea of at-home cholesterol “hacking” kits raises some intriguing possibilities. As personalized medicine advances, perhaps we will see more accessible tools for monitoring and managing cholesterol. The potential for ‘unexpected enlightenment’ is definitely a bonus!
Editor: MedTechNews.Uk
Thank you to our Sponsor Esdebe