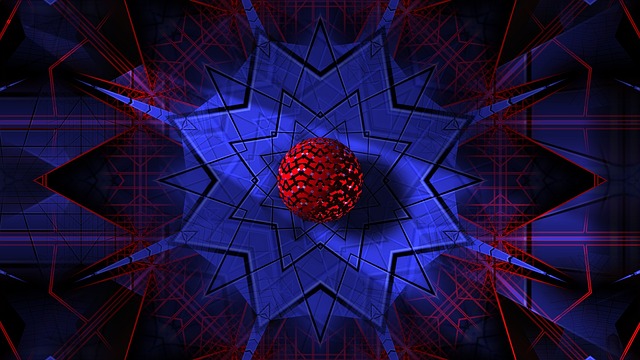
Abstract
Nanoscale materials, characterized by dimensions in the 1-100 nanometer range, exhibit unique physical, chemical, and biological properties that are significantly different from their bulk counterparts. This report provides a comprehensive overview of nanoscale materials, exploring their synthesis, diverse applications across various fields, and the inherent challenges associated with their development and deployment. We delve into the specific properties of a novel nanoscale material, nTG-DFP-COF, designed for illuminating cancer cells during cryosurgery, analyzing its chemical composition, synthesis process, biocompatibility, toxicity, fluorescence mechanism, stability, and potential for large-scale production. Furthermore, we examine other applications of nanoscale materials in cancer diagnosis and treatment, including drug delivery, imaging, and photothermal therapy, alongside a discussion of regulatory considerations for their use in medicine. The report concludes by highlighting future research directions and the transformative potential of nanoscale materials in revolutionizing various sectors, particularly healthcare.
Many thanks to our sponsor Esdebe who helped us prepare this research report.
1. Introduction
The field of nanotechnology has witnessed exponential growth in recent decades, driven by the unique properties and versatile applications of nanoscale materials. These materials, with at least one dimension in the range of 1-100 nanometers, bridge the gap between atomic and macroscopic scales, exhibiting size-dependent characteristics that are not observed in bulk materials. Quantum mechanical effects become prominent at this scale, leading to altered electronic, optical, magnetic, and catalytic properties. This size-dependent behavior enables the design and fabrication of materials with tailored functionalities for a wide range of applications, spanning from electronics and energy to medicine and environmental science [1].
Nanoscale materials can be broadly classified into several categories, including nanoparticles, nanowires, nanotubes, nanofilms, and quantum dots. Each category possesses distinct properties and applications. For instance, nanoparticles, such as gold nanoparticles (AuNPs) and iron oxide nanoparticles (Fe3O4 NPs), are widely used in drug delivery and imaging due to their high surface area and ease of functionalization. Nanowires and nanotubes, composed of materials like silicon or carbon, exhibit exceptional electrical and mechanical properties, making them suitable for use in sensors, transistors, and composite materials. Nanofilms, thin layers of material deposited on a substrate, are employed in coatings, displays, and solar cells. Quantum dots, semiconductor nanocrystals, exhibit size-tunable fluorescence and are used in bioimaging and displays [2].
The development of nanoscale materials necessitates sophisticated synthesis techniques that enable precise control over size, shape, and composition. Various methods have been developed, including chemical synthesis, physical vapor deposition, and self-assembly techniques. Chemical synthesis involves the use of chemical reactions to form nanoscale materials from precursor molecules. Physical vapor deposition involves the vaporization of a material and its subsequent deposition onto a substrate. Self-assembly techniques involve the spontaneous organization of molecules or nanoparticles into ordered structures. Each technique has its advantages and disadvantages, depending on the desired material properties and application [3].
This report aims to provide a comprehensive overview of nanoscale materials, encompassing their synthesis, properties, applications, and future directions. Special emphasis will be placed on a novel nanoscale material, nTG-DFP-COF, designed for illuminating cancer cells during cryosurgery. We will delve into the specific properties of this material, including its chemical composition, synthesis process, biocompatibility, toxicity profile, fluorescence mechanism, stability, and potential for large-scale production. Furthermore, we will explore other applications of nanoscale materials in cancer diagnosis and treatment and discuss the regulatory aspects of their use in medicine.
Many thanks to our sponsor Esdebe who helped us prepare this research report.
2. Synthesis and Characterization of Nanoscale Materials
2.1. Synthesis Methods
The synthesis of nanoscale materials is a critical step in determining their properties and applications. Several methods have been developed, each with its own advantages and disadvantages. These methods can be broadly categorized into top-down and bottom-up approaches.
2.1.1. Top-Down Approaches:
Top-down approaches involve the breaking down of bulk materials into nanoscale structures. These methods typically involve mechanical or chemical processes to reduce the size of the starting material. Examples of top-down approaches include:
- Mechanical Milling: This involves the use of mechanical forces to grind bulk materials into smaller particles. Ball milling is a common technique where the material is placed in a rotating container with grinding media, such as balls. While relatively simple and scalable, mechanical milling can result in broad particle size distributions and introduce defects into the material [4].
- Etching: This involves the selective removal of material from a bulk substrate using chemical or physical processes. Chemical etching uses chemical solutions to dissolve specific materials, while physical etching uses techniques like ion beam milling to remove material layer by layer. Etching can produce well-defined nanoscale structures but often requires complex masking and patterning techniques [5].
- Lithography: This involves the use of light or electron beams to pattern a resist layer on a substrate, followed by etching or deposition processes to create nanoscale structures. Lithography is widely used in the microelectronics industry to fabricate integrated circuits but can also be adapted for the fabrication of nanoscale materials. However, lithography is typically limited to two-dimensional structures and can be expensive and time-consuming [6].
2.1.2. Bottom-Up Approaches:
Bottom-up approaches involve the building of nanoscale materials from atoms or molecules. These methods typically involve chemical reactions or self-assembly processes to create the desired structures. Examples of bottom-up approaches include:
- Chemical Synthesis: This involves the use of chemical reactions to form nanoscale materials from precursor molecules. Chemical synthesis can produce highly uniform and monodisperse nanoparticles with controlled size, shape, and composition. Examples include sol-gel synthesis, co-precipitation, and hydrothermal synthesis [7].
- Vapor Deposition: This involves the vaporization of a material and its subsequent deposition onto a substrate. Physical vapor deposition (PVD) techniques, such as sputtering and evaporation, and chemical vapor deposition (CVD) techniques, such as plasma-enhanced CVD, are commonly used to grow thin films and nanowires [8].
- Self-Assembly: This involves the spontaneous organization of molecules or nanoparticles into ordered structures. Self-assembly is driven by interparticle interactions, such as van der Waals forces, electrostatic interactions, and hydrogen bonding. Self-assembly can produce complex and hierarchical structures but requires careful control over the experimental conditions [9].
2.2. Characterization Techniques
Characterizing nanoscale materials is essential to understand their properties and performance. Various techniques are used to determine the size, shape, composition, and structure of nanoscale materials. These techniques can be broadly categorized into microscopy, spectroscopy, and diffraction methods.
-
Microscopy:
- Scanning Electron Microscopy (SEM): SEM uses a focused beam of electrons to image the surface of a material. SEM can provide high-resolution images of the morphology and size of nanoscale materials [10].
- Transmission Electron Microscopy (TEM): TEM uses a beam of electrons that passes through a thin sample to create an image. TEM can provide information about the internal structure and composition of nanoscale materials at the atomic level [11].
- Atomic Force Microscopy (AFM): AFM uses a sharp tip to scan the surface of a material and measure the forces between the tip and the surface. AFM can provide information about the topography, mechanical properties, and electrical properties of nanoscale materials [12].
-
Spectroscopy:
-
X-ray Photoelectron Spectroscopy (XPS): XPS uses X-rays to excite electrons from a material and measure their kinetic energy. XPS can provide information about the elemental composition and chemical states of nanoscale materials [13].
- Ultraviolet-Visible Spectroscopy (UV-Vis): UV-Vis spectroscopy measures the absorption and transmission of light through a material. UV-Vis spectroscopy can provide information about the optical properties and electronic structure of nanoscale materials [14].
- Raman Spectroscopy: Raman spectroscopy measures the scattering of light by a material. Raman spectroscopy can provide information about the vibrational modes and molecular structure of nanoscale materials [15].
-
Diffraction:
-
X-ray Diffraction (XRD): XRD uses X-rays to diffract from a material and measure the diffraction pattern. XRD can provide information about the crystal structure and lattice parameters of nanoscale materials [16].
Many thanks to our sponsor Esdebe who helped us prepare this research report.
3. nTG-DFP-COF: A Novel Nanoscale Material for Cancer Cell Illumination
3.1. Chemical Composition and Structure
The novel nanoscale material, nTG-DFP-COF, is a fluorescent Covalent Organic Framework (COF) specifically designed for illuminating cancer cells during cryosurgery. COFs are crystalline porous polymers constructed from organic building blocks linked by strong covalent bonds [17]. The “nTG-DFP” nomenclature likely refers to the specific building blocks used in the COF synthesis: “TG” potentially represents a triazine-based building block, and “DFP” may denote a derivative of diphenylphosphine oxide. The exact chemical structures of these building blocks are crucial for understanding the COF’s properties. The COF structure provides a rigid framework that can encapsulate and stabilize fluorescent molecules, enhancing their performance in cryosurgical conditions.
The COF’s porous nature is a critical feature, allowing for the efficient diffusion of cryoprotective agents and the controlled release of therapeutic compounds, if integrated. The pore size and shape can be tailored by selecting different building blocks, offering a means to fine-tune the COF’s properties for specific applications [18]. The high surface area of COFs also allows for efficient loading of fluorophores or other imaging agents.
3.2. Synthesis Process
The synthesis of nTG-DFP-COF likely involves a solvothermal or microwave-assisted reaction between the TG and DFP monomers. The reaction is typically carried out in an organic solvent at elevated temperatures and pressures to promote the formation of covalent bonds and the crystallization of the COF structure. Careful control of the reaction conditions, such as temperature, reaction time, and solvent composition, is essential to obtain high-quality COF crystals with the desired size and morphology [19].
Post-synthetic modification (PSM) may be employed to further enhance the COF’s properties. For example, the COF can be functionalized with targeting ligands that specifically bind to cancer cells. This can be achieved by attaching molecules, such as antibodies or peptides, to the COF’s surface or within its pores [20]. The nanoparticle form (nTG-DFP-COF) is presumably achieved through controlled precipitation or sonication of the bulk COF material. Achieving a narrow size distribution is critical for predictable biodistribution and cellular uptake.
3.3. Biocompatibility and Toxicity Profile
Biocompatibility is a critical consideration for any material intended for biomedical applications. The biocompatibility of nTG-DFP-COF depends on the intrinsic properties of the COF framework and the presence of any functional groups or encapsulated molecules. Ideally, the COF should be non-toxic, non-immunogenic, and biodegradable. Evaluating the cytotoxicity of nTG-DFP-COF using in vitro cell culture assays is essential. These assays assess the viability and proliferation of cells exposed to different concentrations of the material [21].
In vivo studies in animal models are necessary to evaluate the systemic toxicity and biodistribution of nTG-DFP-COF. These studies should assess the accumulation of the material in different organs, as well as any signs of inflammation or tissue damage. The degradation products of the COF should also be non-toxic and easily cleared from the body. Given that phosphine oxides can have varying degrees of toxicity, the choice of DFP derivative is critical, and rigorous toxicity testing is paramount.
3.4. Fluorescence Mechanism
The fluorescence of nTG-DFP-COF at low temperatures is a key feature that enables the visualization of cancer cells during cryosurgery. The fluorescence mechanism is likely related to the interaction between the fluorophore and the COF framework. The COF structure can provide a rigid environment that restricts the movement of the fluorophore, reducing non-radiative decay pathways and enhancing fluorescence intensity. The rigidification of the fluorophore at low temperatures may further enhance its fluorescence by suppressing thermal quenching [22].
The specificity of the fluorescence for cancer cells may be achieved through several mechanisms. First, the COF can be functionalized with targeting ligands that specifically bind to receptors overexpressed on cancer cells. Second, the COF can be designed to be selectively taken up by cancer cells due to their enhanced permeability and retention (EPR) effect. The EPR effect is a phenomenon where nanoparticles preferentially accumulate in tumors due to their leaky vasculature and impaired lymphatic drainage [23].
3.5. Stability and Large-Scale Production
Stability is an important factor for the practical application of nTG-DFP-COF. The COF should be stable in physiological conditions, such as pH, temperature, and ionic strength. The COF should also be stable during storage and handling. The stability of the COF can be improved by modifying its chemical structure or by coating it with a protective layer [24].
Large-scale production of nTG-DFP-COF is essential for its widespread use. The synthesis process should be scalable, cost-effective, and reproducible. The use of inexpensive and readily available starting materials is also important. The process parameters, such as temperature, pressure, and reaction time, should be optimized to maximize the yield and quality of the COF. Continuous flow synthesis methods may be employed to achieve large-scale production [25].
Many thanks to our sponsor Esdebe who helped us prepare this research report.
4. Applications of Nanoscale Materials in Cancer Diagnosis and Treatment
Nanoscale materials have revolutionized cancer diagnosis and treatment due to their unique properties, including high surface area, tunable size, and enhanced permeability and retention (EPR) effect. These properties enable targeted drug delivery, improved imaging, and novel therapeutic modalities.
4.1. Drug Delivery
Nanoparticles can be used to encapsulate and deliver drugs directly to cancer cells, minimizing off-target effects and improving therapeutic efficacy. Nanoparticles can be functionalized with targeting ligands that specifically bind to receptors overexpressed on cancer cells, further enhancing their selectivity [26]. Liposomes, polymeric nanoparticles, and mesoporous silica nanoparticles are commonly used for drug delivery [27].
4.2. Imaging
Nanoparticles can be used as contrast agents for various imaging modalities, such as MRI, CT, and PET. Nanoparticles can enhance the sensitivity and resolution of these imaging techniques, allowing for the early detection and accurate staging of cancer. Quantum dots, gold nanoparticles, and iron oxide nanoparticles are commonly used for imaging [28]. The use of near-infrared (NIR) emitting nanoparticles is particularly advantageous due to their deep tissue penetration [29].
4.3. Photothermal Therapy
Photothermal therapy (PTT) involves the use of nanoparticles to convert light energy into heat, which can selectively kill cancer cells. Nanoparticles, such as gold nanoparticles and carbon nanotubes, can efficiently absorb light in the near-infrared region and generate heat upon irradiation [30]. PTT is a minimally invasive and highly targeted therapeutic approach.
4.4. Other Therapies
- Photodynamic Therapy (PDT): Nanoparticles can deliver photosensitizers to tumor sites, where light activation generates reactive oxygen species, leading to cell death [31].
- Gene Therapy: Nanoparticles can be used to deliver therapeutic genes to cancer cells, correcting genetic defects or enhancing immune responses [32].
- Immunotherapy: Nanoparticles can be used to stimulate the immune system to recognize and destroy cancer cells [33].
Many thanks to our sponsor Esdebe who helped us prepare this research report.
5. Regulatory Aspects of Nanoscale Materials in Medicine
The use of nanoscale materials in medicine is subject to strict regulatory oversight to ensure their safety and efficacy. The Food and Drug Administration (FDA) in the United States and the European Medicines Agency (EMA) in Europe are responsible for regulating the development and approval of nanomedicines. The regulatory process for nanomedicines is complex and requires extensive preclinical and clinical data [34].
Key regulatory considerations include:
- Characterization: Thorough characterization of the nanoscale material is essential, including its size, shape, composition, surface properties, and stability. The characterization data should be reproducible and well-documented [35].
- Biocompatibility and Toxicity: Comprehensive biocompatibility and toxicity studies are required to assess the safety of the nanoscale material. These studies should evaluate the potential for acute and chronic toxicity, immunogenicity, and genotoxicity [36].
- Pharmacokinetics and Biodistribution: Detailed pharmacokinetic and biodistribution studies are needed to understand the absorption, distribution, metabolism, and excretion of the nanoscale material. These studies should assess the accumulation of the material in different organs and tissues [37].
- Efficacy: Clinical trials are required to demonstrate the efficacy of the nanomedicine in treating the target disease. These trials should be well-designed and controlled and should include appropriate endpoints [38].
The regulatory landscape for nanomedicines is constantly evolving, and developers must stay abreast of the latest guidelines and requirements. Collaboration between researchers, industry, and regulatory agencies is essential to facilitate the safe and effective development of nanomedicines [39].
Many thanks to our sponsor Esdebe who helped us prepare this research report.
6. Future Directions and Conclusion
Nanoscale materials hold immense promise for revolutionizing various fields, particularly healthcare. Future research directions include:
- Development of novel synthesis methods: Developing more efficient and scalable synthesis methods that allow for precise control over the size, shape, and composition of nanoscale materials.
- Design of multifunctional nanoparticles: Designing nanoparticles with multiple functionalities, such as drug delivery, imaging, and therapy, to achieve synergistic effects.
- Personalized nanomedicine: Tailoring nanomedicines to individual patients based on their genetic profile and disease characteristics.
- Translation of nanomedicines to the clinic: Accelerating the translation of nanomedicines from the laboratory to the clinic by addressing regulatory hurdles and optimizing manufacturing processes.
In conclusion, nanoscale materials offer a unique opportunity to address critical challenges in cancer diagnosis and treatment. The development of novel materials, such as nTG-DFP-COF, and the continued exploration of their diverse applications will undoubtedly lead to significant advancements in healthcare. However, careful consideration of safety, efficacy, and regulatory aspects is essential to ensure the responsible and ethical development of nanomedicines.
Many thanks to our sponsor Esdebe who helped us prepare this research report.
References
[1] Poole, C. P., & Owens, F. J. (2003). Introduction to nanotechnology. John Wiley & Sons.
[2] Ratner, M. A., & Ratner, D. (2002). Nanotechnology: A gentle introduction to the next big idea. Prentice Hall Professional.
[3] Buzea, C., Pacheco, I. I., & Robbie, K. (2007). Nanomaterials and nanoparticles: Sources and toxicity. Biointerphases, 2(4), MR17-MR71.
[4] Suryanarayana, C. (2001). Mechanical alloying and milling. Progress in materials science, 46(1-2), 1-184.
[5] Madou, M. J. (2018). Fundamentals of microfabrication and nanotechnology. CRC press.
[6] Jaeger, R. C. (2002). Introduction to microelectronic fabrication. Prentice Hall.
[7] Brinker, C. J., & Scherer, G. W. (2013). Sol-gel science: the physics and chemistry of sol-gel processing. Academic press.
[8] Ohring, M. (2001). Materials science of thin films. Academic press.
[9] Whitesides, G. M., & Grzybowski, B. (2002). Self-assembly at all scales. Science, 295(5564), 2418-2421.
[10] Goldstein, J. I., Newbury, D. E., Michael, J. R., Ritchie, N. W. M., Turnbull, D. C., & Fiori, C. (2017). Scanning electron microscopy and X-ray microanalysis. Springer.
[11] Williams, D. B., & Carter, C. B. (2009). Transmission electron microscopy: a textbook for materials science. Springer.
[12] Bhushan, B. (2017). Introduction to tribology. John Wiley & Sons.
[13] Briggs, D., & Seah, M. P. (Eds.). (1990). Practical surface analysis: Auger and X-ray photoelectron spectroscopy. John Wiley & Sons.
[14] Skoog, D. A., Holler, F. J., & Crouch, S. R. (2017). Principles of instrumental analysis. Cengage learning.
[15] Long, D. A. (2002). The Raman effect: a unified treatment of the theory of Raman scattering by molecules. John Wiley & Sons.
[16] Cullity, B. D., & Stock, S. R. (2001). Elements of X-ray diffraction. Prentice Hall.
[17] Cote, A. P., Benin, A. I., Ockwig, N. W., O’Keeffe, M., Matzger, A. J., Yaghi, O. M. (2005). Porous, crystalline, covalent organic frameworks. Science, 310(5751), 1166-1170.
[18] Diercks, C. S., & Yaghi, O. M. (2017). The chemistry of covalent organic frameworks. Science, 355(6328), eaal1585.
[19] Waller, D., & Yaghi, O. M. (2015). Covalent organic frameworks. Chemical Society Reviews, 44(11), 3469-3527.
[20] Feng, X., Ding, X., & Jiang, D. (2012). Covalent organic frameworks. Chemical Society Reviews, 41(18), 6010-6022.
[21] Nel, A., Madler, L., Velegol, D., Xia, T., Hoek, E. M., Somasundaran, P., … & Thompson, M. (2009). Understanding biophysicochemical interactions at the nano–bio interface. Nature materials, 8(7), 543-557.
[22] Lakowicz, J. R. (2006). Principles of fluorescence spectroscopy. Springer Science & Business Media.
[23] Maeda, H., Wu, J., Sawa, T., Matsumura, Y., & Hori, K. (2000). Tumor vascular permeability and the EPR effect in macromolecular therapeutics: a review. Journal of controlled release, 65(1-2), 271-284.
[24] Khan, I., Saeed, K., & Khan, I. (2019). Nanoparticles: Properties, applications and toxicities. Arabian Journal of Chemistry, 12(7), 908-931.
[25] Baumann, M., & Albertus, P. (2021). Continuous manufacturing of nanoparticles: Current state and future trends. Chemical Engineering & Technology, 44(1), 29-41.
[26] Ferrari, M. (2005). Cancer nanotechnology: opportunities and challenges. Nature Reviews Cancer, 5(3), 161-171.
[27] Allen, T. M., & Cullis, P. R. (2004). Drug delivery systems: entering the mainstream. Science, 303(5665), 1818-1822.
[28] Weissleder, R. (2001). Scaling down imaging: molecular sensors for in vivo diagnostics. Nature biotechnology, 19(4), 316-317.
[29] Hong, G., Diao, S., Chang, J., Antaris, A. L., & Dai, H. (2014). Carbon nanotubes for in vivo imaging and cancer therapy. Chemical reviews, 115(19), 10816-10906.
[30] Huang, X., Jain, P. K., El-Sayed, I. H., & El-Sayed, M. A. (2007). Gold nanoparticles: interesting optical properties and recent applications in cancer diagnostics and therapy. Nanomedicine, 2(5), 681-693.
[31] Castano, A. P., Mroz, P., Hamblin, M. R. (2006). Photodynamic therapy and anti-tumour immunity. Nature Reviews Cancer, 6(7), 535-545.
[32] Anderson, W. F. (1998). Human gene therapy. Nature, 392(6679 Suppl), 25-30.
[33] Irvine, D. J., Swartz, M. A., & Hubbell, J. A. (2013). Materials for cancer immunotherapy. Nature materials, 12(11), 978-990.
[34] Ryman-Rasmussen, J. P., Riviere, J. E., & Monteiro-Riviere, N. A. (2007). Current regulatory status of engineered nanomaterials. Journal of nanobiotechnology, 5(1), 1-9.
[35] ISO/TS 80004-1:2010 Nanotechnologies — Vocabulary — Part 1: Core terms
[36] Oberdörster, G., Oberdörster, E., & Oberdörster, J. (2005). Nanotoxicology: an emerging discipline evolving from studies of ultrafine particles. Environmental health perspectives, 113(7), 823-839.
[37] Alexis, F., Pridgen, E., Molnar, L. K., & Farokhzad, O. C. (2008). Factors affecting the in vivo performance of nanomedicines. Chemistry & biology, 15(10), 1016-1025.
[38] Ventola, C. L. (2012). The nanomedicine revolution: part 1: emerging concepts. Pharmacy and Therapeutics, 37(9), 512.
[39] Wagner, V., Dullaart, A., Bock, A. K., & Zweck, A. (2006). The emerging nanomedicine landscape. Nature biotechnology, 24(10), 1211-1217.
So, nTG-DFP-COF illuminates cancer cells during cryosurgery, huh? Does this mean glow-in-the-dark tumors will be the next fashion statement at the oncology ward? Perhaps a matching line of fluorescent bandages?
That’s quite a thought! While glowing tumors aren’t the goal, your comment highlights the potential for enhanced visualization during procedures. The fluorescence allows surgeons to precisely target and remove cancer cells, potentially leading to better outcomes. Maybe less “fashion statement” and more “precision medicine”!
Editor: MedTechNews.Uk
Thank you to our Sponsor Esdebe
So, nTG-DFP-COF lights up cancer cells during cryosurgery! I wonder, could we adapt it to highlight other cell types? Imagine using this technology to guide stem cell therapies or even illuminate those pesky neurons in Alzheimer’s research. The possibilities seem as limitless as the nano-world itself.
That’s a fantastic point! The potential to adapt this for other cell types, like in stem cell therapies or Alzheimer’s research, is something we’re actively exploring. The key is modifying the targeting ligands to bind to specific cell surface markers. The future is bright, and your insight is spot on!
Editor: MedTechNews.Uk
Thank you to our Sponsor Esdebe
Illuminating cancer during cryosurgery? So next we will have nanoscale materials for early diagnosis? Imagine a world where a simple injection could flag cancerous cells years before traditional methods! That’s the *real* sci-fi dream.
That’s a great vision! Early diagnosis is definitely a key goal. We’re also exploring using these materials to improve the accuracy of biopsies, enabling doctors to pinpoint suspicious areas with greater confidence. Imagine combining early detection with precise intervention! What other applications do you see?
Editor: MedTechNews.Uk
Thank you to our Sponsor Esdebe