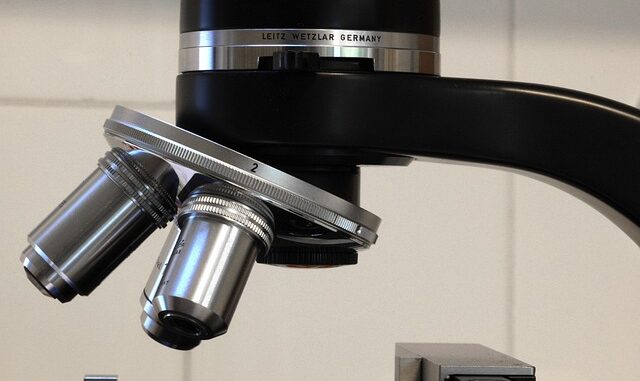
Abstract
Organ-on-a-chip (OoC) technology has emerged as a transformative approach in biomedical research, offering a physiologically relevant platform for studying human biology and disease. These microfluidic devices, engineered to mimic the structure and function of human organs, are poised to revolutionize drug discovery, toxicology testing, and personalized medicine. This review delves into the technical aspects of OoC models, exploring their design principles, fabrication methods, and diverse applications. We discuss the incorporation of relevant cell types, extracellular matrix components, and microenvironmental cues to recapitulate the complexities of native tissues. We analyze the advantages and limitations of OoCs compared to traditional cell cultures and animal models, highlighting their potential to reduce animal testing and improve the predictive power of preclinical studies. Furthermore, we showcase specific examples of OoC applications in disease modeling, drug screening, and regenerative medicine. Finally, we address the current challenges and future directions of this rapidly evolving field, emphasizing the need for standardization, validation, and integration with other omics technologies to unlock the full potential of OoC technology.
Many thanks to our sponsor Esdebe who helped us prepare this research report.
1. Introduction
The field of biomedical research has long sought methods to accurately replicate human physiology in vitro. Traditional cell culture techniques, while valuable, often fall short in capturing the intricate architecture, cellular interactions, and dynamic microenvironment of living tissues. Animal models, although more complex, suffer from interspecies differences that limit their translatability to human outcomes. Organ-on-a-chip (OoC) technology has emerged as a promising alternative, offering a microengineered platform that bridges the gap between simplified in vitro assays and complex in vivo systems [1].
OoCs, also known as microphysiological systems (MPS), are microfluidic devices designed to mimic the structure and function of human organs at the cellular level. These devices typically incorporate living cells cultured in a microenvironment that replicates key aspects of the organ’s native physiology, such as three-dimensional (3D) architecture, fluid flow, mechanical forces, and biochemical gradients [2]. By recreating these physiological conditions, OoCs provide a more realistic and predictive platform for studying human biology, disease mechanisms, and drug responses. The technology has seen rapid advancement in recent years, and the range of physiological processes that can be studied using OoC devices has expanded rapidly.
The potential applications of OoC technology are vast, spanning diverse areas of biomedical research, including drug discovery, toxicology testing, disease modeling, and personalized medicine. In drug discovery, OoCs can be used to screen drug candidates for efficacy and toxicity in a more human-relevant context, potentially reducing the attrition rate of drugs in clinical trials. In toxicology testing, OoCs can provide a more sensitive and predictive assessment of the effects of chemicals and environmental toxins on human health, potentially reducing the reliance on animal testing. In disease modeling, OoCs can be used to recreate the complex pathophysiology of human diseases, such as cancer, cardiovascular disease, and neurodegenerative disorders, enabling the development of novel therapeutic strategies. In personalized medicine, OoCs can be used to test the effectiveness of different treatments on patient-derived cells, potentially tailoring therapy to individual needs.
This research report aims to provide a comprehensive overview of OoC technology, encompassing its design principles, fabrication methods, applications, and future directions. We will explore the technical challenges and opportunities associated with OoC development, highlighting the need for standardization, validation, and integration with other advanced technologies to fully realize the potential of this transformative approach.
Many thanks to our sponsor Esdebe who helped us prepare this research report.
2. Design and Fabrication of Organ-on-a-Chip Devices
The design and fabrication of OoC devices involve a multidisciplinary approach, integrating principles from microfluidics, cell biology, materials science, and engineering. A typical OoC device consists of one or more microchannels, typically ranging from tens to hundreds of micrometers in width and height, fabricated on a biocompatible substrate. These microchannels are designed to mimic the microvasculature of human organs, allowing for precise control of fluid flow, nutrient delivery, and waste removal [3].
2.1. Materials and Microfabrication Techniques
The choice of materials for OoC fabrication is crucial, as they must be biocompatible, transparent for imaging, and amenable to microfabrication techniques. Polydimethylsiloxane (PDMS) is a widely used material due to its biocompatibility, optical transparency, and ease of microfabrication using soft lithography. Soft lithography involves creating a master mold with the desired microchannel patterns using techniques such as photolithography or micromachining. PDMS is then poured onto the mold, cured, and peeled off to create a replica of the microchannel patterns. The PDMS replica is then bonded to a glass or PDMS substrate to form a closed microfluidic channel [4].
Other materials used for OoC fabrication include thermoplastics, such as polymethylmethacrylate (PMMA) and polycarbonate (PC), which offer advantages in terms of cost-effectiveness and scalability. These materials can be microfabricated using techniques such as micromilling, laser ablation, and injection molding. Hydrogels, such as collagen, fibrin, and Matrigel, are also commonly used to create a 3D cell culture environment within the OoC device. These hydrogels can be patterned using microfabrication techniques to create complex tissue architectures [5].
More recent advances include the integration of stimuli-responsive polymers that alter their properties in response to external cues like temperature or pH, enabling dynamic control over the chip’s environment and cellular behaviour. This allows for the simulation of time-dependent processes or the induction of specific cellular responses.
2.2. Microchannel Design and Fluid Flow Control
The design of microchannels is critical for replicating the microenvironment of human organs. The dimensions of the microchannels, the spacing between them, and the geometry of the channel network all influence the fluid flow patterns, shear stress levels, and nutrient gradients within the OoC device. Computational fluid dynamics (CFD) simulations are often used to optimize the microchannel design and predict the flow behavior [6].
Precise control of fluid flow is essential for maintaining cell viability, delivering nutrients, removing waste products, and mimicking the hemodynamic forces experienced by cells in the body. Microfluidic pumps, such as syringe pumps and peristaltic pumps, are used to deliver fluids to the OoC device at controlled flow rates. Microvalves and microswitches can be integrated into the OoC device to control the flow direction and timing of fluid delivery [7]. The use of microfluidic feedback loops has also emerged, allowing for real-time adjustments to flow rates based on cellular activity or metabolic consumption within the chip.
2.3. Integration of Sensors and Actuators
Integration of sensors and actuators into OoC devices allows for real-time monitoring and control of the microenvironment. Sensors can be used to measure parameters such as temperature, pH, oxygen concentration, glucose levels, and cell viability. Actuators, such as microheaters, micropumps, and microvalves, can be used to control the temperature, fluid flow, and chemical gradients within the OoC device [8].
Electrochemical sensors, optical sensors, and mechanical sensors are commonly used in OoC devices. Electrochemical sensors can be used to measure the concentration of ions and metabolites. Optical sensors can be used to measure cell viability, proliferation, and differentiation. Mechanical sensors can be used to measure the forces exerted by cells or the stiffness of the extracellular matrix. The integration of these sensors and actuators enables closed-loop control of the microenvironment, allowing for precise manipulation of cellular behavior and tissue development.
Many thanks to our sponsor Esdebe who helped us prepare this research report.
3. Cell Sources and Tissue Engineering in Organ-on-a-Chip Models
Reproducing the cell-cell interactions, tissue architecture, and extracellular matrix (ECM) composition of human organs is paramount for creating physiologically relevant OoC models. The choice of cell sources and the techniques used to engineer tissues within the OoC device are crucial for achieving this goal.
3.1. Cell Sources for Organ-on-a-Chip Models
A variety of cell sources can be used for OoC models, including primary cells, immortalized cell lines, and stem cells. Primary cells, isolated directly from human or animal tissues, offer the advantage of representing the native cell phenotype and function. However, primary cells can be difficult to obtain, maintain in culture, and may exhibit batch-to-batch variability. Immortalized cell lines, derived from cancer cells or genetically modified to proliferate indefinitely, offer a more readily available and reproducible cell source. However, immortalized cell lines may exhibit altered phenotypes and functions compared to primary cells [9].
Stem cells, including embryonic stem cells (ESCs) and induced pluripotent stem cells (iPSCs), offer the potential to generate a wide range of differentiated cell types for OoC models. iPSCs, generated by reprogramming adult somatic cells, offer the advantage of being patient-specific, enabling the development of personalized OoC models for drug testing and disease modeling. However, stem cell differentiation protocols can be complex and time-consuming, and the resulting differentiated cells may not fully recapitulate the phenotype and function of mature cells [10].
Recent advances focus on co-culturing multiple cell types within the OoC to better represent the cellular diversity within organs. This allows researchers to study cell-cell interactions and their impact on overall tissue function.
3.2. Tissue Engineering Strategies for Organ-on-a-Chip Models
Tissue engineering techniques are used to create 3D tissue structures within OoC devices. These techniques include cell seeding, bioprinting, and self-assembly. Cell seeding involves introducing cells into a scaffold material, such as a hydrogel, and allowing them to adhere and proliferate. Bioprinting involves using a robotic system to deposit cells and biomaterials in a layer-by-layer fashion to create complex tissue architectures. Self-assembly involves using the intrinsic properties of cells and ECM components to guide the formation of tissue structures [11].
Hydrogels are commonly used as scaffold materials in OoC models due to their biocompatibility, water content, and ability to mimic the ECM. The ECM is a complex network of proteins and polysaccharides that provides structural support and biochemical cues to cells. Mimicking the ECM composition and architecture is crucial for replicating the native tissue microenvironment in OoC models. Researchers often use decellularized ECM (dECM) from native tissues or synthetic ECM components to create more physiologically relevant scaffolds. dECM retains the complex protein composition and architecture of the original tissue, providing a more natural environment for cell growth and differentiation [12].
Furthermore, the incorporation of mechanical stimuli, such as cyclic stretch or fluid shear stress, is essential for replicating the dynamic mechanical environment of certain organs. These mechanical stimuli can influence cell behavior, tissue development, and disease progression [13].
3.3. Mimicking the Organ-Specific Microenvironment
Achieving organ-specific functionality in OoC models requires recreating the unique microenvironmental cues of each organ. This includes replicating the specific cell types, ECM composition, biochemical gradients, and mechanical forces that are characteristic of each organ. For example, a liver-on-a-chip model would need to incorporate hepatocytes, sinusoidal endothelial cells, and Kupffer cells, as well as the specific ECM components found in the liver, such as collagen and laminin. The model would also need to recreate the hepatic sinusoidal architecture and the flow of blood through the liver [14].
A lung-on-a-chip model would need to incorporate alveolar epithelial cells and endothelial cells, as well as the specific ECM components found in the lung, such as elastin and fibronectin. The model would also need to recreate the air-liquid interface and the mechanical forces associated with breathing [15]. Similarly, a brain-on-a-chip would require neurons, astrocytes, and microglia, along with a matrix mimicking the brain’s unique biochemical environment and specialized vasculature. The ability to precisely control these variables sets OoCs apart from traditional methods.
Many thanks to our sponsor Esdebe who helped us prepare this research report.
4. Advantages and Limitations of Organ-on-a-Chip Models
OoC technology offers several advantages over traditional cell culture and animal models, but also faces some limitations that need to be addressed for its widespread adoption.
4.1. Advantages Over Traditional Cell Culture and Animal Models
OoC models offer several advantages over traditional cell culture and animal models. First, OoC models provide a more physiologically relevant in vitro environment that better mimics the structure and function of human organs. This allows for a more accurate assessment of drug efficacy and toxicity, potentially reducing the attrition rate of drugs in clinical trials [16].
Second, OoC models can be used to study human biology and disease mechanisms in a more controlled and ethical manner than animal models. OoC models can be used to generate human-specific data, eliminating the interspecies differences that limit the translatability of animal data to human outcomes. This can significantly reduce the need for animal testing, addressing ethical concerns and reducing costs [17].
Third, OoC models allow for real-time monitoring and control of the microenvironment, enabling researchers to study dynamic cellular processes and responses to stimuli. This level of control is not possible with traditional cell culture or animal models. This real-time monitoring is often coupled with sophisticated analysis tools, providing insights into cellular behaviour at an unprecedented level.
Fourth, OoC models can be miniaturized and automated, allowing for high-throughput screening of drug candidates and chemicals. This can significantly accelerate the drug discovery process and reduce the cost of drug development [18]. The ability to create arrays of these chips enables efficient screening of multiple drug candidates and treatment conditions in parallel.
4.2. Limitations and Challenges of Organ-on-a-Chip Models
Despite their advantages, OoC models also face several limitations and challenges. First, OoC models are complex to design, fabricate, and operate, requiring specialized expertise and equipment. This can limit their accessibility to researchers who are not familiar with microfluidics and tissue engineering [19].
Second, OoC models may not fully recapitulate the complexity of human organs, lacking the full complement of cell types, ECM components, and physiological cues that are present in vivo. This can limit the predictive power of OoC models for certain applications. Specifically, the lack of a fully functional immune system representation is a major limitation for applications involving inflammation or immune-mediated diseases.
Third, OoC models can be difficult to validate and standardize, making it challenging to compare results across different laboratories and studies. Standardization of OoC protocols, materials, and data analysis methods is needed to ensure reproducibility and comparability [20].
Fourth, the cost of developing and using OoC models can be high, especially for complex models that require specialized materials and equipment. This can limit their adoption by smaller research groups and companies.
Fifth, challenges remain in scaling up OoC technology for industrial applications. While individual chips are effective for research, manufacturing large numbers of consistent and reliable chips for high-throughput screening or diagnostic purposes is complex.
Many thanks to our sponsor Esdebe who helped us prepare this research report.
5. Applications of Organ-on-a-Chip Technology
OoC technology has found applications in a wide range of biomedical research areas, including drug discovery, toxicology testing, disease modeling, and personalized medicine.
5.1. Drug Discovery and Development
OoCs are increasingly used in drug discovery to improve the efficiency and success rate of preclinical studies. They enable researchers to screen drug candidates for efficacy and toxicity in a more human-relevant context, predicting drug responses more accurately than traditional in vitro assays or animal models. For example, a liver-on-a-chip model can be used to assess the hepatotoxicity of drug candidates, identifying compounds that may cause liver damage in humans [21].
OoCs can also be used to study the pharmacokinetics and pharmacodynamics of drugs, providing insights into how drugs are absorbed, distributed, metabolized, and excreted by the body. This information is crucial for optimizing drug dosage and delivery [22]. Furthermore, OoCs allow for the study of drug-drug interactions, which are a major concern in polypharmacy.
5.2. Toxicology Testing
OoCs offer a promising alternative to animal testing for assessing the toxicity of chemicals, cosmetics, and environmental toxins. They provide a more sensitive and predictive assessment of the effects of these substances on human health, potentially reducing the reliance on animal testing. For example, a lung-on-a-chip model can be used to assess the respiratory toxicity of inhaled chemicals, identifying compounds that may cause lung damage or respiratory irritation [23].
OoCs can also be used to study the mechanisms of toxicity, providing insights into how chemicals interact with cells and tissues to cause adverse effects. This information can be used to develop safer chemicals and products. The integration of advanced analytical techniques with OoC platforms, such as metabolomics and proteomics, allows for a comprehensive assessment of toxicological effects at the molecular level.
5.3. Disease Modeling
OoCs can be used to recreate the complex pathophysiology of human diseases, such as cancer, cardiovascular disease, and neurodegenerative disorders. These models can be used to study disease mechanisms, identify drug targets, and test the effectiveness of novel therapeutic strategies. For example, a cancer-on-a-chip model can be used to study tumor growth, invasion, and metastasis, providing insights into the mechanisms of cancer progression [24]. In the context of cancer research, microfluidic devices are used to simulate the tumor microenvironment and examine the interaction between tumor cells and immune cells to understand how the body combats a tumor. These microfluidic devices have improved the evaluation of cancer therapeutics by mimicking aspects of cancer at the micro-scale.
OoCs can also be used to model genetic diseases, such as cystic fibrosis and muscular dystrophy, by incorporating cells derived from patients with these conditions. This allows for the study of disease-specific mechanisms and the development of personalized therapies [25].
5.4. Personalized Medicine
OoCs have the potential to revolutionize personalized medicine by enabling the testing of different treatments on patient-derived cells. This allows for tailoring therapy to individual needs, improving treatment outcomes and reducing adverse effects. For example, an iPSC-derived heart-on-a-chip model can be used to test the effectiveness of different antiarrhythmic drugs on patient-specific cardiomyocytes, identifying the most effective drug for each patient [26].
OoCs can also be used to predict patient response to chemotherapy, allowing for the selection of the most effective chemotherapy regimen for each patient. This can improve the efficacy of cancer treatment and reduce the risk of drug resistance.
Many thanks to our sponsor Esdebe who helped us prepare this research report.
6. Future Directions and Challenges
OoC technology is a rapidly evolving field with significant potential to transform biomedical research and healthcare. However, several challenges need to be addressed to fully realize its potential.
6.1. Standardization and Validation
Standardization and validation of OoC protocols, materials, and data analysis methods are crucial for ensuring reproducibility and comparability across different laboratories and studies. Standardized protocols for cell culture, microfabrication, and data acquisition are needed to minimize variability and ensure the reliability of OoC results. Validation studies are needed to demonstrate the accuracy and predictive power of OoC models for specific applications [27].
Community-driven initiatives, such as the Microphysiological Systems Database (MPS-DB), are playing a critical role in promoting standardization and data sharing in the field. The development of standardized reference materials and quality control procedures is also essential for ensuring the reliability of OoC technology.
6.2. Integration with Omics Technologies
Integration of OoC technology with other advanced technologies, such as genomics, proteomics, and metabolomics, can provide a more comprehensive understanding of cellular responses and disease mechanisms. Combining OoC models with omics technologies allows for the identification of biomarkers, drug targets, and disease mechanisms that may not be apparent using traditional methods [28].
For example, transcriptomic analysis of cells cultured in an OoC model can reveal changes in gene expression in response to drug treatment or disease conditions. Proteomic analysis can identify changes in protein expression and post-translational modifications. Metabolomic analysis can identify changes in metabolic pathways and metabolite levels. Integrating these omics data with OoC data can provide a more holistic view of cellular behavior and disease processes.
6.3. Complexity and Physiological Relevance
Efforts are needed to increase the complexity and physiological relevance of OoC models. This includes incorporating a wider range of cell types, ECM components, and physiological cues, as well as replicating the dynamic interactions between different organs and tissues. Multi-organ-on-a-chip models, which connect multiple OoC devices to simulate the interactions between different organs, are emerging as a promising approach for studying systemic effects and drug metabolism [29].
The development of more sophisticated microfabrication techniques is needed to create more complex tissue architectures and recreate the intricate microvasculature of human organs. The use of computational modeling and simulation can also help to optimize the design and operation of OoC models.
6.4. Scalability and Cost-Effectiveness
Improving the scalability and cost-effectiveness of OoC technology is essential for its widespread adoption in industry and academia. Developing more efficient microfabrication techniques, reducing the cost of materials and reagents, and automating OoC operation can help to lower the cost of OoC models. Developing high-throughput OoC platforms that can screen large numbers of drug candidates or chemicals in parallel can also improve the efficiency of drug discovery and toxicology testing. Furthermore, research into 3D printing methods for chip fabrication offers promise for customized, low-cost production.
6.5. Regulatory Acceptance and Clinical Translation
Achieving regulatory acceptance and clinical translation of OoC technology is a key goal for the field. Regulatory agencies, such as the FDA and EMA, are actively evaluating the potential of OoC models for drug approval and toxicology testing. Demonstrating the reliability and predictive power of OoC models for specific applications is essential for gaining regulatory acceptance [30].
Clinical translation of OoC technology will require the development of robust and reproducible OoC models that can be used to predict patient response to treatment. The use of patient-derived cells and personalized OoC models is essential for achieving this goal. Successful clinical translation of OoC technology has the potential to revolutionize personalized medicine and improve patient outcomes.
Many thanks to our sponsor Esdebe who helped us prepare this research report.
7. Conclusion
Organ-on-a-chip technology has emerged as a transformative approach in biomedical research, offering a physiologically relevant platform for studying human biology and disease. OoC models have the potential to revolutionize drug discovery, toxicology testing, disease modeling, and personalized medicine. While challenges remain in terms of standardization, validation, and scalability, the field is rapidly advancing, and OoC technology is poised to play an increasingly important role in the future of biomedical research and healthcare.
Many thanks to our sponsor Esdebe who helped us prepare this research report.
References
[1] Bhatia, S. N., & Ingber, D. E. (2014). Microfluidic organs-on-chips. Nature biotechnology, 32(8), 760-772.
[2] Esch, E. W., Bahinski, A., & Roth, A. (2015). Organs-on-chips: designing integrated microdevices for drug discovery and development. Drug discovery today: technologies, 12(1-2), e1-e5.
[3] Halldorsson, S., Lateef, M. A., Berger, A., & Ruzicka, J. (2015). Organs-on-chips: a future platform for drug development. Applied in vitro toxicology, 1(3), 151-166.
[4] Quake, S. R., & Scherer, A. (2000). From micro-to nanofabrication with soft materials. Science, 290(5496), 1536-1540.
[5] Tibbitt, M. W., & Anseth, K. S. (2009). Hydrogels as extracellular matrix mimics for 3D cell culture. Biotechnology and bioengineering, 103(4), 655-663.
[6] Kim, H. J., Huh, D., Hamilton, G., & Ingber, D. E. (2012). Human gut-on-a-chip inhabited by microbial flora that experiences intestinal peristalsis-like motions. Lab on a Chip, 12(12), 2167-2174.
[7] Beebe, D. J., Mensing, G. A., & Walker, G. M. (2002). Microfluidic tectonics: a comprehensive construction platform for microfluidic systems. Annual review of biomedical engineering, 4(1), 261-286.
[8] Madou, M. J., Zoval, J. V., Stenger, D. A., & Coulombe, T. A. (2006). Lab on a chip for environmental and biological sensing. Annual review of analytical chemistry, 1(1), 143-179.
[9] Masters, T. A., & Shah, R. N. (2011). Choosing cell types for 3D culture: critical considerations. Expert opinion on drug discovery, 6(10), 1031-1042.
[10] Rossi, G., Manfrin, A., & Lutolf, M. P. (2018). Engineering organoids. Materials today, 21(3), 236-249.
[11] Khademhosseini, A., & Langer, R. (2016). A decade of progress in tissue engineering. Nature reviews materials, 1(3), 1-17.
[12] Gilbert, T. W., Sellaro, T. L., & Badylak, S. F. (2006). Decellularization of tissues and organs. Biomaterials, 27(19), 3675-3683.
[13] Parker, K. K., & Ingber, D. E. (2011). Microfluidic heart-on-a-chip devices. Nature nanotechnology, 6(6), 369-376.
[14] Edington, C. D., Kuti, J. L., Hammond, R. A., Kurtz, C. L., Jones, D. E., Gerber, A. C., … & Griffith, L. G. (2015). In vitro human liver model with integrated microfluidic biosensor for real-time assessment of liver health and function. Lab on a Chip, 15(2), 647-657.
[15] Huh, D., Matthews, B. D., Mammoto, A., Montoya-Zavala, M., Hsin, H. Y., & Ingber, D. E. (2010). Reconstituting organ-level lung functions on a chip. Science, 328(5986), 1662-1668.
[16] Low, L. A., Tagle, D. A., & Austin, C. P. (2011). Microphysiological systems for drug development. Trends in biotechnology, 29(12), 604-611.
[17] Marx, U., Grillberger, L., у. А., & Gülden, M. (2016). Alternatives to animal testing: state-of-the-art and future perspectives. Archives of toxicology, 90(9), 2099-2199.
[18] Oleaga, C., Verrells, F. E., Bernabini, C., Browne, C. D., & Elvassore, N. (2016). Multi-organ-on-a-chip for systemic ADME/Tox assessment. Drug discovery today, 21(2), 323-329.
[19] Bhatia, S. N. (2015). Tissue engineering: the microscale advantage. Science translational medicine, 7(278), 278ps6-278ps6.
[20] Pamies, D., Alépée, N., Bal-Price, A., Grinberg, L., Hartung, T., Holmdahl, R., … & Smirnova, L. (2014). Intra-laboratory reproducibility of a human embryonic stem cell-based test for developmental neurotoxicity. Toxicology in Vitro, 28(7), 1253-1263.
[21] Ramaiahgari, S. C., den Braver, M. W., Herpers, B., Buijs, M., Horst-Kreft, D., Verpoorte, E., … & van de Stolpe, A. (2014). A microfluidic 3D cell culture system for anti-cancer drug testing. PloS one, 9(6), e98867.
[22] Virag, T., & Hickman, J. J. (2011). Coupling of microelectrode arrays and microfluidic devices for real-time monitoring of electrophysiological activity in cultured neuronal networks. Journal of neuroscience methods, 196(2), 161-169.
[23] Ehrhardt, C., & Lange-Asschenfeldt, B. (2018). In vitro models of the respiratory tract. Wiley interdisciplinary reviews: nanotechnology and nanomedicine, 10(1), e1478.
[24] Imamura, Y., Kishi, Y., Wang, D., Huynh, Q. T., Clark, A. T., Le, A. Q., … & Eves, P. (2015). Micromachined 3D culture platform for investigating tumor-stroma interaction. Biomaterials, 61, 27-37.
[25] Leclerc, E., Sakai, Y., Fujii, T., & Takei, M. (2019). Disease modeling using organ-on-a-chip technology. Regenerative therapy, 12, 72-82.
[26] Mathur, A., Loskill, P., Shao, K., Ganesan, A., Healy, K. E., & Bers, D. M. (2015). Human iPSC-based cardiac microphysiological system for drug screening applications. Scientific reports, 5, 8883.
[27] Waldmann, T. A., Keun, H. C., Lash, L. H., & Westerhoff, H. V. (2014). Standardization and validation of in vitro toxicity assays. Toxicology and applied pharmacology, 278(3), 259-267.
[28] Wikswo, J. P., Block, F. E., Conrad, C. M., Domach, M. M., Dumbleton, J., Griffith, L. G., … & Yarmush, M. L. (2017). Engineering challenges for instrumenting and controlling integrated organ-on-chip devices. IEEE transactions on biomedical engineering, 64(10), 2234-2250.
[29] Zhang, Y. S., Aleman, J., Shin, S. R., Kilic, T., Kim, D., Khademhosseini, A. (2016). Microfluidic bioprinting for 3D tissue engineering. Biomaterials, 98, 140-162.
[30] Ingber, D. E. (2022). Human organs-on-chips for disease modelling, drug development and personalized medicine. Nature Reviews Genetics, 23(8), 467-491.
Be the first to comment