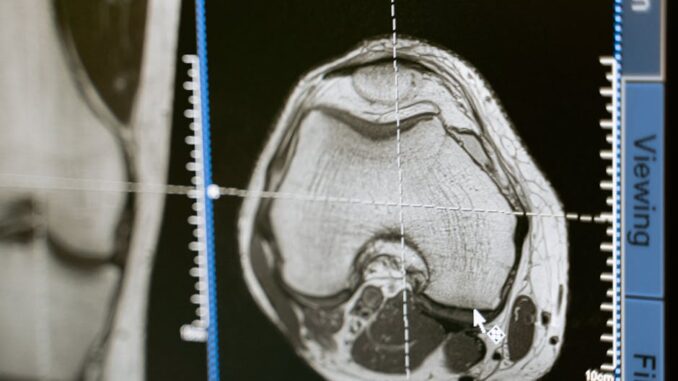
Abstract
Terahertz (THz) technology, encompassing the electromagnetic spectrum between microwaves and infrared light (0.1–10 THz), has emerged as a versatile tool with applications spanning diverse fields, from security and communication to material characterization and biomedicine. This report provides a comprehensive overview of the current state-of-the-art in THz technology, focusing on recent advancements in THz sources, detectors, and imaging systems. We explore the fundamental principles underlying THz interaction with matter, highlighting the unique spectroscopic signatures and imaging capabilities that differentiate THz technology from other modalities. The report delves into specific applications, including non-destructive testing, security screening, high-speed wireless communication, and medical diagnostics. We also address the inherent challenges associated with THz technology, such as atmospheric absorption and limited penetration depth, and discuss potential solutions and future research directions aimed at overcoming these limitations. Finally, we offer a perspective on the long-term prospects of THz technology and its potential to revolutionize various sectors.
Many thanks to our sponsor Esdebe who helped us prepare this research report.
1. Introduction
The terahertz (THz) region of the electromagnetic spectrum, situated between the microwave and infrared regions (typically defined as 0.1 THz to 10 THz, corresponding to wavelengths of 3 mm to 30 μm), has witnessed a surge in research and development over the past few decades. This is primarily driven by the unique properties of THz radiation and its potential applications in a wide range of fields. Unlike X-rays, THz radiation is non-ionizing and considered safe for biological tissues, making it attractive for medical imaging. Moreover, many materials exhibit distinct spectral features in the THz range, enabling the identification and characterization of substances such as explosives, pharmaceuticals, and biological molecules. The coherent nature of THz waves allows for advanced imaging techniques, including THz time-domain spectroscopy (THz-TDS) and THz computed tomography (THz-CT), offering both amplitude and phase information about the sample under investigation.
However, the development of THz technology has been hindered by several challenges. Historically, the “THz gap” existed due to the lack of efficient and compact sources and detectors. Although significant progress has been made in recent years, improving the power, bandwidth, and cost-effectiveness of THz systems remains a crucial area of research. Another major challenge is the strong absorption of THz radiation by water vapor in the atmosphere, which limits the range and resolution of THz imaging and spectroscopy in ambient conditions. Furthermore, the penetration depth of THz radiation is limited in highly conductive materials, such as metals and some biological tissues, restricting its applicability in certain scenarios. This report will delve deeper into these advancements and challenges, offering an expert perspective on the current landscape and future trajectory of THz technology.
Many thanks to our sponsor Esdebe who helped us prepare this research report.
2. Fundamentals of Terahertz Radiation and its Interaction with Matter
2.1 Terahertz Radiation: Properties and Generation
THz radiation, as an electromagnetic wave, exhibits properties characteristic of both radio waves and infrared light. Its relatively long wavelength compared to visible light allows it to penetrate through many non-conducting materials, such as plastics, paper, and textiles, while its frequency is high enough to interact with molecular vibrations and rotations.
Various techniques are employed for generating THz radiation. These can be broadly classified into electronic and photonic approaches. Electronic methods typically involve multiplying the frequency of lower-frequency oscillators, such as Gunn diodes or IMPATT diodes, to reach the THz regime. While these sources are relatively compact and cost-effective, they often suffer from limited power and bandwidth. Photonic approaches, on the other hand, exploit nonlinear optical processes, such as optical rectification and difference frequency generation (DFG), using ultrashort laser pulses. These sources can generate broadband THz radiation with high power and coherence, but they tend to be more complex and expensive. Quantum cascade lasers (QCLs) have also emerged as a promising THz source, offering compact and electrically pumped operation with moderate power levels. Free-electron lasers (FELs) can also be used to generate THz radiation, but these are very large and expensive facilities only available in a small number of laboratories around the world.
2.2 Interaction of Terahertz Radiation with Matter
The interaction of THz radiation with matter is governed by the material’s dielectric properties in the THz frequency range. Polar molecules, such as water, exhibit strong absorption due to rotational transitions. This is why THz radiation is heavily absorbed by atmospheric water vapour, resulting in the need for purged or vacuum systems for applications that require long transmission distances. Crystalline materials and other solids display characteristic absorption and reflection spectra due to lattice vibrations (phonons) and electronic transitions.
One of the most significant advantages of THz spectroscopy is its sensitivity to vibrational modes of molecules. Many organic molecules, including pharmaceuticals, explosives, and biological molecules, exhibit unique vibrational fingerprints in the THz region. This allows for the identification and characterization of these substances through THz spectroscopy or imaging. THz waves also interact with free carriers in semiconductors, making it possible to probe their electronic properties. In the context of biological materials, the interaction of THz waves with water content and protein structures enables the differentiation between healthy and diseased tissues.
2.3 THz Time-Domain Spectroscopy (THz-TDS)
THz-TDS is a powerful spectroscopic technique that measures both the amplitude and phase of the THz radiation transmitted through or reflected from a sample. In a typical THz-TDS system, an ultrashort laser pulse is split into two beams: one to generate THz radiation and the other to detect it. The THz radiation is generated by illuminating a photoconductive antenna or a nonlinear crystal. The transmitted or reflected THz radiation is then detected using another photoconductive antenna or electro-optic crystal, gated by the delayed laser pulse. By measuring the time-domain waveform of the THz pulse, the complex refractive index of the sample can be extracted as a function of frequency using Fourier transformation. THz-TDS offers several advantages over traditional spectroscopic techniques, including high signal-to-noise ratio, broad bandwidth, and the ability to measure both the real and imaginary parts of the dielectric function.
Many thanks to our sponsor Esdebe who helped us prepare this research report.
3. Advancements in Terahertz Sources and Detectors
3.1 Terahertz Sources
Significant progress has been made in recent years in developing more efficient and powerful THz sources. Electronic sources, such as frequency multipliers based on Schottky diodes or heterostructure barrier varactors (HBVs), have been improved to deliver higher output power at higher frequencies. These sources are compact and relatively inexpensive, making them suitable for applications where portability and cost are important considerations. However, they typically suffer from limited bandwidth and frequency tunability. Quantum Cascade Lasers (QCLs) have also experienced a dramatic increase in both power and operating temperature. QCLs are particularly interesting because they offer a compact footprint compared to a free electron laser, but offer a coherent high power output, furthermore QCLs are electrically pumped offering simplicity and ease of integration into a system.
Photonic sources, on the other hand, offer wider bandwidth and higher power levels. Femtosecond lasers are often used in conjunction with nonlinear optical crystals or photoconductive antennas to generate THz radiation. The development of new nonlinear materials with higher conversion efficiencies has led to improved THz power levels. For example, organic crystals, such as DAST (4-N,N-dimethylamino-4′-N’-methyl-stilbazolium tosylate) and DSTMS (4-N,N-dimethylamino-4′-N’-methyl-stilbazolium 2,6-dimethylbenzenesulfonate), exhibit significantly higher nonlinearities compared to inorganic crystals like ZnTe. Photoconductive antennas, fabricated on semiconductor substrates with short carrier lifetimes, can also generate broadband THz radiation when illuminated by femtosecond laser pulses. The development of novel antenna designs and substrate materials has further improved the efficiency and bandwidth of these sources.
3.2 Terahertz Detectors
The development of sensitive and fast THz detectors is crucial for realizing high-performance THz imaging and spectroscopy systems. Various types of THz detectors have been developed, each with its own advantages and limitations. Coherent detectors, such as photoconductive antennas and electro-optic sampling detectors, measure the amplitude and phase of the THz radiation directly. These detectors offer high sensitivity and temporal resolution, making them suitable for THz-TDS applications. However, they typically require complex optical alignment and are sensitive to ambient noise.
Incoherent detectors, such as bolometers and pyroelectric detectors, measure the intensity of the THz radiation. Bolometers are based on the temperature-dependent resistance of a material and can achieve very high sensitivity at cryogenic temperatures. Pyroelectric detectors are based on the change in polarization of a material due to temperature variations and offer relatively fast response times at room temperature. Schottky diodes can also be used as THz detectors, providing a compact and cost-effective solution for power measurements. The performance of THz detectors is often characterized by their noise equivalent power (NEP), which is the minimum detectable power level. Recent advancements in detector technology have focused on reducing the NEP and improving the speed and bandwidth of THz detectors.
3.3 Emerging THz Technologies
Beyond the traditional sources and detectors, emerging technologies are opening new avenues for THz applications. For example, metamaterials, which are artificially engineered materials with subwavelength structures, can be used to manipulate THz radiation in unprecedented ways. Metamaterials can be designed to exhibit negative refractive index, cloaking effects, and enhanced light-matter interactions, enabling the development of novel THz devices such as lenses, filters, and modulators. Another promising area is the development of compact and high-performance THz imaging systems based on focal plane arrays (FPAs). These FPAs consist of a large number of individual THz detectors arranged in a two-dimensional array, allowing for real-time imaging without the need for mechanical scanning. These advances hold great promise for future THz applications.
Many thanks to our sponsor Esdebe who helped us prepare this research report.
4. Applications of Terahertz Technology
4.1 Non-Destructive Testing (NDT) and Industrial Inspection
THz imaging and spectroscopy offer a non-destructive and non-contact method for inspecting materials and structures. THz radiation can penetrate through many opaque materials, such as plastics, ceramics, and composites, allowing for the detection of hidden defects, voids, and delaminations. This makes THz technology particularly useful for quality control in manufacturing industries, such as aerospace, automotive, and electronics. For example, THz imaging can be used to inspect the thickness and uniformity of coatings, to detect cracks and voids in composite materials, and to identify counterfeit pharmaceuticals. THz-TDS can also be used to measure the water content and density of materials, providing valuable information about their structural integrity. Using pulsed THz systems the reflected pulse can be used to find the location of defects within a multi-layered structure, using the change in refractive index at each interface.
4.2 Security Screening
THz imaging has shown great potential for security screening applications. Unlike X-rays, THz radiation is non-ionizing and considered safe for human exposure. THz imaging can be used to detect concealed weapons, explosives, and other contraband hidden under clothing or in packages. The unique spectral signatures of many explosives in the THz range allow for their identification with high specificity. THz security screening systems are being deployed in airports, train stations, and other public venues to enhance security measures. However, challenges remain in improving the speed and resolution of THz imaging systems and in developing robust algorithms for automatic threat detection.
4.3 Wireless Communication
The THz frequency range offers a vast untapped bandwidth for wireless communication. The increasing demand for higher data rates and bandwidth has driven research into THz communication systems. THz communication has the potential to provide data rates exceeding 100 Gbps, enabling new applications such as wireless data centers, high-definition video streaming, and virtual reality. However, several challenges need to be addressed before THz communication can become a reality. These include developing efficient THz transceivers, overcoming atmospheric absorption, and mitigating the effects of multipath fading. Researchers are exploring various techniques, such as beam steering, channel equalization, and adaptive modulation, to improve the performance of THz communication systems. Advances in THz antenna design, modulation techniques, and coding schemes are also crucial for realizing the full potential of THz communication.
4.4 Biomedical Applications
THz imaging and spectroscopy hold great promise for biomedical applications, including disease diagnostics, drug discovery, and personalized medicine. The non-ionizing nature of THz radiation makes it safe for imaging biological tissues. THz imaging can be used to differentiate between healthy and diseased tissues based on their different dielectric properties in the THz range. For example, THz imaging has shown potential for detecting skin cancer, breast cancer, and other types of cancer. THz-TDS can also be used to monitor the hydration levels of skin, providing valuable information for dermatological applications. In addition, THz spectroscopy can be used to study the interaction of drugs with biological molecules, accelerating the drug discovery process. The ability to probe biological materials in a non-destructive and label-free manner makes THz technology an attractive tool for biomedical research. However, the high water content of tissue means the penetration depth of THz radiation is severely limited. This can be overcome by collecting reflected THz waves instead of transmitted waves.
Many thanks to our sponsor Esdebe who helped us prepare this research report.
5. Challenges and Future Directions
5.1 Atmospheric Absorption
The strong absorption of THz radiation by water vapor in the atmosphere is a major challenge for THz applications. This absorption limits the range and resolution of THz imaging and spectroscopy in ambient conditions. Several strategies have been developed to mitigate the effects of atmospheric absorption, including purging the THz system with dry nitrogen or operating it in a vacuum. However, these solutions are often impractical or expensive. Another approach is to use frequency windows where atmospheric absorption is minimal. Researchers are also exploring the use of THz waveguides and integrated THz circuits to reduce the path length of THz radiation in the atmosphere. The development of more powerful THz sources and more sensitive detectors can also help to overcome atmospheric absorption.
5.2 Penetration Depth
The penetration depth of THz radiation is limited in highly conductive materials, such as metals and some biological tissues. This restricts the applicability of THz technology in certain scenarios. Several techniques have been developed to improve the penetration depth of THz radiation, including using lower frequencies, optimizing the angle of incidence, and employing contrast agents. Metamaterials can also be used to enhance the penetration of THz radiation into materials. In the context of medical imaging, researchers are exploring the use of THz endoscopes to access internal organs. The development of more powerful THz sources and more sensitive detectors can also help to improve the penetration depth.
5.3 Cost and Complexity
THz systems are often expensive and complex, limiting their widespread adoption. The high cost is primarily due to the sophisticated components required, such as femtosecond lasers, nonlinear crystals, and high-speed detectors. Efforts are underway to reduce the cost and complexity of THz systems by developing more compact and integrated THz sources and detectors. The use of CMOS technology and other low-cost manufacturing techniques can help to reduce the cost of THz devices. The development of user-friendly software and interfaces can also make THz systems easier to operate and maintain.
5.4 Future Research Directions
Future research in THz technology will focus on addressing the challenges mentioned above and on exploring new applications. Areas of active research include:
- Developing more powerful and efficient THz sources: This includes exploring new materials and device designs for QCLs, frequency multipliers, and photoconductive antennas.
- Improving the sensitivity and speed of THz detectors: This includes developing new bolometers, pyroelectric detectors, and Schottky diodes with improved performance.
- Developing new THz imaging techniques: This includes exploring new modalities such as THz computed tomography (THz-CT) and THz near-field microscopy.
- Exploring the use of metamaterials for THz applications: This includes designing new metamaterials for THz lenses, filters, modulators, and sensors.
- Developing integrated THz circuits and systems: This includes integrating THz sources, detectors, and other components onto a single chip.
- Exploring new applications of THz technology: This includes investigating the use of THz radiation for environmental monitoring, agriculture, and fundamental science.
Many thanks to our sponsor Esdebe who helped us prepare this research report.
6. Conclusion
Terahertz technology has emerged as a powerful tool with applications spanning diverse fields. Recent advancements in THz sources, detectors, and imaging systems have significantly expanded the capabilities of this technology. While challenges remain, such as atmospheric absorption and limited penetration depth, ongoing research efforts are addressing these limitations. The development of more compact, efficient, and cost-effective THz systems will pave the way for widespread adoption in various industries. The future of THz technology is bright, with the potential to revolutionize areas such as non-destructive testing, security screening, wireless communication, and biomedical diagnostics. As research continues to push the boundaries of THz technology, we can expect to see even more innovative applications emerge in the years to come.
Many thanks to our sponsor Esdebe who helped us prepare this research report.
References
- Tonouchi, M. (2007). Cutting-edge terahertz technology. Nature Photonics, 1(2), 97-105.
- Dhillon, S. S., Vitiello, M. S., Linfield, E. H., Davies, A. G., Hoffmann, M. C., Booske, J., … & Zhang, X. C. (2017). The 2017 terahertz science and technology roadmap. Journal of Physics D: Applied Physics, 50(4), 043001.
- Lee, Y. S. (2009). Principles of terahertz science and technology. Springer Science & Business Media.
- Jepsen, P. U., Cooke, D. G., & Koch, M. (2011). Terahertz spectroscopy and imaging–modern techniques and applications. Laser & Photonics Reviews, 5(1), 124-166.
- Fitzgerald, A. J., & Woodward, R. M. (2008). Progress in terahertz imaging: potential for clinical applications. Biomedical Imaging and Intervention Journal, 4(1), e5.
- Yang, X., Zhao, X., Dai, J., & Zhang, X. C. (2007). Terahertz wave imaging. TrAC Trends in Analytical Chemistry, 26(7), 628-636.
- Rogalski, A., Antoszewski, J., & Faraone, L. (2009). Third-generation infrared photodetector arrays. Journal of Applied Physics, 105(12), 121101.
- Woolard, D. L., Brown, E. R., Pepper, M., Kemp, M., Crowe, T. W., Choi, J., … & Strano, M. S. (2011). The interdisciplinary demand for THz sensing: identifying the opportunities. Proceedings of the IEEE, 99(7), 1152-1159.
- Globus, T. R., Woolard, D. L., Crowe, T. W., Gelmont, B. L., Hess, K., Samoilenko, V., … & Bykov, Y. V. (2001). THz-range compatibility of nitrogen-purged systems: Advantages for sensor, diagnostic, and analytic applications. Sensor Review, 21(4), 269-282.
- Watts, C. M., Padilla, W. J., Highstrete, J. J., Reinhard, M., Lee, R., & Taylor, A. J. (2012). Metamaterial electromagnetic cloak at terahertz frequencies. Nature materials, 11(5), 392-396.
- Fukunaga, K., Ozeki, Y., & Kawase, K. (2017). Development of terahertz imaging systems and their application to non-destructive testing. Sensors, 17(2), 325.
- Nagai, K., Tanaka, M., Ohtake, H., Murakami, M., Tonouchi, M., Shibasaki, K., & Nakajima, M. (2011). Development of terahertz security screening system with transmission/reflection dual imaging. Japanese Journal of Applied Physics, 50(9R), 09MB01.
- Kleine-Ostmann, T., & Nagatsuma, T. (2011). A perspective on terahertz communications. Journal of Infrared, Millimeter, and Terahertz Waves, 32(2), 143-171.
- Ashworth, P. C., Pickwell-MacPherson, E., & Miles, R. E. (2009). THz technology for biomedical applications. Optics and Photonics News, 20(11), 32-39.
- Woodward, R. M., Cole, B. E., Taday, P. F., Kemp, M. C., & Fitzgerald, A. J. (2003). Terahertz pulse imaging of ex vivo basal cell carcinoma. Journal of Biomedical Optics, 8(3), 409-417.
- Knobloch, C., Schildknecht, C., Deninger, A., Hemberger, J., & Loidl, A. (2002). Terahertz time-domain spectroscopy of bovine skin: In vitro measurements of the influence of hydration. Journal of Physics D: Applied Physics, 35(24), 3159.
- Singh, S., & Singh, A. (2022). Terahertz Technology: A Review on Recent Advancements, Applications, and Open Challenges. Electronics, 11(15), 2382.
So, THz tech can see through stuff! Can we finally find my keys that vanished into another dimension? Asking for, uh, science!
That’s a fantastic point! While keys to other dimensions might be a *bit* beyond current capabilities, THz technology is revolutionizing non-destructive testing. Imagine using it to find structural weaknesses in bridges or even hidden layers in paintings! What hidden things do you think THz could reveal?
Editor: MedTechNews.Uk
Thank you to our Sponsor Esdebe
So, THz can differentiate between healthy and diseased tissue? Does this mean we’ll soon have a Star Trek-style tricorder for instant health scans? Imagine skipping the waiting room and just getting scanned on the spot!