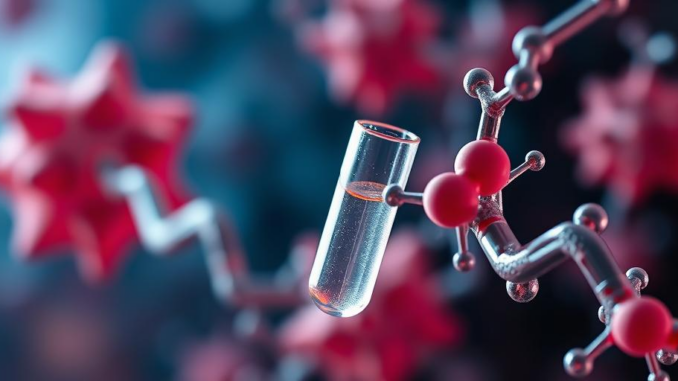
The Evolving Landscape of Insulin: From Metabolic Regulator to Pleiotropic Signaling Hub
Abstract
Insulin, historically recognized as the key regulator of glucose homeostasis, is now understood to possess a much broader range of physiological functions. This report delves into the multifaceted roles of insulin, extending beyond its well-established metabolic effects to encompass its influence on cell growth, differentiation, inflammation, and even cognitive function. We examine the intricacies of insulin production and secretion, exploring the underlying molecular mechanisms and regulatory pathways involved. Furthermore, we critically assess the impact of insulin resistance, not only in the context of type 2 diabetes but also in other metabolic disorders and age-related conditions. The report also analyzes the latest advancements in insulin-based therapies, including innovative insulin analogs and delivery systems, as well as non-pharmacological strategies for maintaining insulin sensitivity and improving metabolic health. Finally, we propose future directions for research, highlighting the need for a more holistic understanding of insulin signaling and its implications for human health and disease.
1. Introduction: Beyond Glucose Homeostasis
For nearly a century, insulin has been celebrated as the hormone indispensable for controlling blood glucose levels. Its discovery and subsequent therapeutic use revolutionized the treatment of type 1 diabetes mellitus (T1DM), transforming a fatal condition into a manageable chronic illness. However, restricting the role of insulin solely to glucose metabolism provides an incomplete picture of its complex biological activity. It is now clear that insulin acts as a crucial signaling molecule involved in a diverse array of physiological processes, including cell growth and differentiation, lipid metabolism, protein synthesis, and even neuronal function [1, 2]. These pleiotropic effects are mediated through a complex network of intracellular signaling pathways initiated by the binding of insulin to its receptor (IR), a receptor tyrosine kinase (RTK), located on the surface of target cells.
The classical view of insulin action involves the stimulation of glucose uptake into skeletal muscle and adipose tissue, primarily through the translocation of the glucose transporter GLUT4 to the plasma membrane [3]. In the liver, insulin promotes glycogen synthesis and inhibits gluconeogenesis. These actions collectively contribute to the lowering of blood glucose levels following food intake. However, insulin also exerts significant effects on lipid metabolism, promoting lipogenesis and inhibiting lipolysis in adipose tissue, thereby influencing the storage and utilization of fatty acids [4]. Moreover, insulin stimulates protein synthesis and inhibits protein degradation in various tissues, contributing to overall anabolic state.
Recent research has expanded our understanding of insulin’s influence beyond traditional metabolic tissues. Insulin receptors are expressed in the brain, where insulin plays a role in regulating neuronal function, synaptic plasticity, and cognitive processes [5]. Disruption of insulin signaling in the brain has been implicated in the pathogenesis of neurodegenerative diseases such as Alzheimer’s disease [6]. Furthermore, insulin signaling has been shown to modulate immune function, influencing inflammatory responses and the activity of immune cells [7]. These findings highlight the importance of considering insulin as a systemic signaling molecule with far-reaching effects on various organ systems.
2. Insulin Production and Secretion: A Multi-Step Process
Insulin is synthesized and secreted by specialized cells called beta cells, located within the islets of Langerhans in the pancreas. The production of insulin is a tightly regulated process involving several key steps, beginning with the transcription of the insulin gene and culminating in the exocytosis of mature insulin granules [8].
The initial step in insulin production is the transcription of the insulin gene (INS) into preproinsulin mRNA. This process is regulated by a variety of transcription factors that are responsive to glucose and other metabolic signals. The preproinsulin mRNA is then translated into a single polypeptide chain called preproinsulin. Preproinsulin contains a signal peptide that directs the protein to the endoplasmic reticulum (ER), where the signal peptide is cleaved off, generating proinsulin [9].
Proinsulin consists of three domains: the B-chain, the C-peptide, and the A-chain. Within the ER, proinsulin undergoes folding and disulfide bond formation, facilitated by chaperone proteins. Proinsulin is then transported to the Golgi apparatus, where it is packaged into secretory granules. Within these granules, proinsulin is cleaved by prohormone convertases (PC1/3 and PC2) and carboxypeptidase E (CPE), resulting in the formation of mature insulin and C-peptide [10].
The mature insulin molecule consists of the A-chain and the B-chain linked by disulfide bonds. Insulin is stored in secretory granules until a stimulus, such as elevated blood glucose levels, triggers its release. Glucose-stimulated insulin secretion (GSIS) is a complex process involving glucose uptake by the beta cell, followed by its metabolism, leading to an increase in intracellular ATP levels. This rise in ATP inhibits ATP-sensitive potassium (KATP) channels, causing membrane depolarization. Depolarization activates voltage-gated calcium channels, leading to an influx of calcium ions into the beta cell. The increase in intracellular calcium triggers the fusion of insulin-containing granules with the plasma membrane and the subsequent release of insulin into the bloodstream [11].
Other factors besides glucose can also modulate insulin secretion. Amino acids, fatty acids, and hormones such as glucagon-like peptide-1 (GLP-1) and glucose-dependent insulinotropic polypeptide (GIP) can stimulate insulin release [12]. These factors act through various signaling pathways to enhance calcium influx and/or increase the sensitivity of the secretory machinery to calcium. Conversely, hormones such as somatostatin and epinephrine can inhibit insulin secretion. Autoimmune destruction of beta cells leads to T1DM, whereas progressive beta cell dysfunction is a prominent feature of T2DM. Therefore, maintaining beta cell health and function is crucial for preventing and treating diabetes.
3. The Insulin Receptor and Downstream Signaling Pathways
Insulin exerts its diverse biological effects by binding to the insulin receptor (IR), a transmembrane receptor tyrosine kinase (RTK) expressed on the surface of many cell types. The IR is a heterotetrameric protein consisting of two alpha subunits and two beta subunits linked by disulfide bonds. The alpha subunits are located extracellularly and are responsible for insulin binding, while the beta subunits contain a tyrosine kinase domain [13].
Upon insulin binding, the IR undergoes autophosphorylation on tyrosine residues within the intracellular domain of the beta subunits. This autophosphorylation activates the tyrosine kinase activity of the IR, enabling it to phosphorylate intracellular substrate proteins, primarily insulin receptor substrate (IRS) proteins. IRS proteins are a family of adaptor proteins that mediate many of the downstream effects of insulin signaling [14].
The phosphorylation of IRS proteins creates docking sites for other signaling molecules, including phosphatidylinositol 3-kinase (PI3K) and growth factor receptor-bound protein 2 (Grb2). Activation of PI3K leads to the production of phosphatidylinositol-3,4,5-trisphosphate (PIP3), which recruits downstream signaling molecules such as Akt (protein kinase B) to the plasma membrane. Akt, once activated by phosphorylation, phosphorylates a variety of target proteins, including glycogen synthase kinase-3 (GSK-3), mammalian target of rapamycin (mTOR), and FOXO transcription factors. These downstream targets mediate the effects of insulin on glucose metabolism, protein synthesis, cell growth, and survival [15].
The Grb2-Sos-Ras-MAPK pathway is another important signaling cascade activated by insulin. Grb2 binds to phosphorylated IRS proteins, recruiting Sos, a guanine nucleotide exchange factor that activates Ras. Activated Ras then initiates a cascade of protein kinases, ultimately leading to the activation of mitogen-activated protein kinases (MAPKs). The MAPK pathway plays a role in cell growth, differentiation, and gene expression [16].
It is important to note that insulin signaling is highly regulated by various feedback mechanisms. For example, the activity of IRS proteins can be negatively regulated by serine/threonine kinases such as mTOR and S6K, which are activated by insulin itself. Furthermore, protein tyrosine phosphatases (PTPs) can dephosphorylate the IR and IRS proteins, thereby terminating insulin signaling [17]. Dysregulation of these feedback mechanisms can contribute to insulin resistance and the development of metabolic disorders.
4. Insulin Resistance: A Metabolic Crossroads
Insulin resistance is a condition in which cells become less responsive to the effects of insulin, requiring higher-than-normal levels of insulin to achieve the same biological effect. Insulin resistance is a central feature of T2DM, but it is also implicated in a wide range of other metabolic disorders, including obesity, metabolic syndrome, non-alcoholic fatty liver disease (NAFLD), and cardiovascular disease [18].
The underlying mechanisms of insulin resistance are complex and multifactorial. Several factors contribute to the development of insulin resistance, including genetic predisposition, obesity, physical inactivity, inflammation, and aging. In obesity, excess accumulation of lipids in tissues such as muscle and liver can interfere with insulin signaling. Lipids, such as diacylglycerol (DAG) and ceramides, can activate serine/threonine kinases that phosphorylate IRS proteins on serine residues, thereby inhibiting their ability to be phosphorylated on tyrosine residues by the IR [19]. This impaired IRS phosphorylation reduces the activation of downstream signaling pathways, leading to decreased glucose uptake and utilization.
Chronic inflammation also plays a role in insulin resistance. Pro-inflammatory cytokines, such as tumor necrosis factor-alpha (TNF-α) and interleukin-6 (IL-6), can activate signaling pathways that interfere with insulin signaling. These cytokines can also promote lipolysis in adipose tissue, leading to an increase in circulating free fatty acids, which further contributes to insulin resistance [20].
In addition, aging is associated with a decline in insulin sensitivity. Several factors contribute to age-related insulin resistance, including decreased muscle mass, increased visceral adiposity, and impaired mitochondrial function [21]. Epigenetic modifications, such as DNA methylation and histone modifications, may also play a role in age-related insulin resistance.
Insulin resistance has profound metabolic consequences. In the liver, insulin resistance impairs the suppression of glucose production, leading to increased hepatic glucose output and hyperglycemia. In muscle, insulin resistance impairs glucose uptake and utilization, contributing to hyperglycemia and decreased glycogen storage. In adipose tissue, insulin resistance impairs the suppression of lipolysis, leading to increased circulating free fatty acids, which further exacerbates insulin resistance in other tissues [22].
The pancreas attempts to compensate for insulin resistance by increasing insulin secretion. However, over time, the beta cells can become exhausted, leading to a decline in insulin production and the development of T2DM. Insulin resistance is also associated with increased risk of cardiovascular disease, likely due to its effects on lipid metabolism, inflammation, and endothelial function. Improving insulin sensitivity through lifestyle interventions such as diet and exercise, as well as through pharmacological interventions, is crucial for preventing and managing metabolic disorders.
5. Advancements in Insulin-Based Therapies
The development of insulin therapy has been a cornerstone in the management of diabetes. However, conventional insulin formulations have limitations, including the risk of hypoglycemia, weight gain, and the need for multiple daily injections. To address these limitations, significant advancements have been made in insulin-based therapies, including the development of novel insulin analogs and delivery systems.
Insulin analogs are genetically engineered insulins with altered amino acid sequences that modify their pharmacokinetic properties. Rapid-acting insulin analogs, such as lispro, aspart, and glulisine, have a faster onset and shorter duration of action compared to regular human insulin. These analogs are designed to more closely mimic the physiological insulin response to meals, reducing the risk of postprandial hyperglycemia and hypoglycemia [23].
Long-acting insulin analogs, such as glargine, detemir, and degludec, provide a more stable and prolonged basal insulin coverage compared to intermediate-acting insulins. These analogs have a reduced risk of nocturnal hypoglycemia and allow for more flexible dosing schedules [24].
In addition to novel insulin analogs, advancements in insulin delivery systems have also improved the management of diabetes. Insulin pens provide a convenient and discreet method for injecting insulin. Insulin pumps, also known as continuous subcutaneous insulin infusion (CSII) devices, deliver a continuous basal infusion of insulin and allow for bolus doses to be administered before meals. Insulin pumps can improve glycemic control and reduce the frequency of hypoglycemic episodes compared to multiple daily injections [25].
Closed-loop insulin delivery systems, also known as artificial pancreas systems, combine a continuous glucose monitor (CGM) with an insulin pump and a control algorithm. The CGM measures blood glucose levels in real-time, and the control algorithm adjusts the insulin delivery rate based on the glucose readings. These systems have shown promise in improving glycemic control and reducing the burden of diabetes management [26].
In addition to subcutaneous insulin delivery, other routes of insulin administration are being investigated, including inhaled insulin, oral insulin, and transdermal insulin. Inhaled insulin provides a rapid onset of action but has been associated with pulmonary side effects. Oral insulin has the potential to provide a more physiological route of insulin delivery but faces challenges related to poor bioavailability due to degradation in the gastrointestinal tract. Transdermal insulin delivery is a non-invasive approach that involves the absorption of insulin through the skin, but achieving adequate insulin absorption remains a challenge [27].
6. Non-Pharmacological Strategies for Maintaining Insulin Sensitivity
While insulin-based therapies are essential for managing diabetes, non-pharmacological strategies play a crucial role in preventing and treating insulin resistance. Lifestyle interventions, such as diet and exercise, are effective in improving insulin sensitivity and reducing the risk of developing T2DM [28].
A healthy diet, rich in fruits, vegetables, whole grains, and lean protein, can improve insulin sensitivity and promote weight loss. Limiting the intake of processed foods, sugary beverages, and saturated fats is also important. Specific dietary patterns, such as the Mediterranean diet and the Dietary Approaches to Stop Hypertension (DASH) diet, have been shown to improve insulin sensitivity and reduce the risk of cardiovascular disease [29].
Regular physical activity is a powerful tool for improving insulin sensitivity. Both aerobic exercise and resistance training have been shown to increase glucose uptake and utilization in muscle. Exercise also promotes weight loss, reduces inflammation, and improves cardiovascular health [30].
In addition to diet and exercise, other lifestyle factors can also influence insulin sensitivity. Adequate sleep is essential for maintaining metabolic health. Sleep deprivation has been linked to insulin resistance, increased appetite, and weight gain [31]. Stress management techniques, such as meditation and yoga, can also improve insulin sensitivity by reducing the levels of stress hormones, such as cortisol [32].
Emerging evidence suggests that the gut microbiome may also play a role in insulin sensitivity. Certain gut bacteria can produce metabolites, such as short-chain fatty acids, that improve insulin sensitivity. Dietary interventions that promote the growth of beneficial gut bacteria, such as a high-fiber diet, may have a positive impact on insulin sensitivity [33].
7. Future Directions and Concluding Remarks
Our understanding of insulin signaling has evolved significantly over the past century, revealing its pleiotropic roles beyond glucose homeostasis. While insulin-based therapies remain a cornerstone of diabetes management, further research is needed to develop more effective and personalized treatment strategies. Future research should focus on several key areas:
- Elucidating the complex interactions between insulin signaling and other signaling pathways. Understanding how insulin signaling interacts with other pathways, such as those involving growth factors, cytokines, and hormones, is crucial for developing targeted therapies that can modulate insulin sensitivity without causing unintended side effects.
- Identifying novel targets for improving insulin sensitivity. Further research is needed to identify novel molecular targets that can be modulated to improve insulin sensitivity. This includes exploring the role of non-coding RNAs, epigenetic modifications, and other emerging factors in insulin resistance.
- Developing more sophisticated insulin delivery systems. Closed-loop insulin delivery systems hold great promise for improving glycemic control and reducing the burden of diabetes management. Future research should focus on developing more accurate and reliable CGMs, as well as more sophisticated control algorithms.
- Investigating the role of insulin signaling in non-metabolic tissues. The role of insulin signaling in the brain, immune system, and other non-metabolic tissues is an area of growing interest. Further research is needed to understand how insulin signaling in these tissues contributes to overall health and disease.
- Personalizing insulin therapy based on individual characteristics. Factors such as genetics, lifestyle, and the gut microbiome can influence insulin sensitivity. Future research should focus on developing personalized insulin therapy strategies that take these factors into account.
In conclusion, insulin is a critical signaling molecule with far-reaching effects on metabolism and overall health. A comprehensive understanding of insulin signaling, its dysregulation in insulin resistance, and the latest advancements in insulin-based therapies is essential for preventing and managing metabolic disorders. By pursuing future research in the areas outlined above, we can continue to improve the lives of individuals with diabetes and other metabolic diseases.
References
[1] Wilcox, G. (2005). Insulin and insulin resistance. Clinical Biochemist Reviews, 26(2), 19.
[2] De Meyts, P. (2016). The insulin receptor: structure, signalling and therapeutic implications. Nature Reviews Endocrinology, 12(2), 93-102.
[3] Leto, D., & Saltiel, A. R. (2012). Regulation of glucose transport by insulin: traffic control of GLUT4. Nature Reviews Molecular Cell Biology, 13(6), 383-396.
[4] Czech, M. P. (2017). Insulin action and resistance: lessons from molecular physiology. American Journal of Physiology-Endocrinology and Metabolism, 312(6), E749-E757.
[5] Kullmann, S., & Heni, M. (2018). Insulin action in the human brain. Journal of Clinical Investigation, 128(4), 1223-1234.
[6] Talbot, K., Wang, H. Y., Holmes, C., Yang, F., Lee, J. H., Gundersen, B. B., … & Trojanowski, J. Q. (2012). Demonstrated brain insulin resistance in Alzheimer’s disease patients. Journal of Clinical Investigation, 122(4), 1316-1338.
[7] Akashi, K., Nagafuchi, S., Sakaguchi, K., Niizuma, M., Funamoto, T., Takizawa, T., … & Takada, H. (2003). Insulin regulates macrophage activation and function. Diabetes, 52(7), 1739-1745.
[8] Steiner, D. F., & Oyer, P. E. (1967). The biosynthesis of insulin and a probable precursor of insulin by a human islet cell adenoma. Proceedings of the National Academy of Sciences, 57(2), 473-480.
[9] Rhodes, C. J., & White, M. F. (2002). Molecular insights into insulin action and insulin resistance: the roles of IRS proteins and AS160. European Journal of Biochemistry, 269(10), 2443-2455.
[10] Steiner, D. F., Smeekens, S. P., Ohagi, S., & Chan, S. J. (1992). The new enzymology of precursor processing endoproteases. Journal of Biological Chemistry, 267(34), 23435-23438.
[11] Henquin, J. C. (2000). Triggering and amplifying pathways of glucose-induced insulin secretion in pancreatic β-cells. Diabetes, 49(11), 1751-1760.
[12] Campbell, J. E., & Drucker, D. J. (2015). Pharmacology, physiology, and mechanisms of incretin hormone action. Cell Metabolism, 21(6), 737-758.
[13] Hubbard, S. R. (1997). Crystal structure of the kinase domain of the human insulin receptor. Nature, 387(6636), 872-881.
[14] White, M. F. (1998). IRS proteins and the insulin signaling network. Molecular and Cellular Biochemistry, 182(1-2), 3-11.
[15] Manning, B. D., & Toker, A. (2017). AKT/PKB signaling: navigating the network. Cell, 169(3), 381-405.
[16] Yoon, S., & Seger, R. (2006). The extracellular signal-regulated kinase: mechanism of activation, biological function, and regulation. Growth Factors, 24(1), 21-44.
[17] Tonks, N. K. (2006). Protein tyrosine phosphatases: from housekeeping enzymes to master regulators. Biochimica et Biophysica Acta (BBA)-Molecular Cell Research, 1763(1), 1-13.
[18] Samuel, V. T., & Shulman, G. I. (2018). Mechanisms for insulin resistance: common threads and missing links. Cell, 175(3), 735-791.
[19] Petersen, M. C., & Shulman, G. I. (2018). Mechanisms of insulin action and insulin resistance. Physiological Reviews, 98(4), 2133-2223.
[20] Hotamisligil, G. S. (2017). Inflammation and metabolic disease. Nature, 542(7640), 177-185.
[21] Dillin, A., Gottschling, D. E., & Nyström, T. (2002). The cell biology of aging. Cold Spring Harbor Perspectives in Biology, 4(5), a010425.
[22] DeFronzo, R. A. (1988). The triumvirate: β-cell, muscle, liver. A collusion responsible for NIDDM. Diabetes, 37(6), 667-687.
[23] Heinemann, L., Linke, A., Rave, K., & Hompesch, B. (1993). Time-action profile of the soluble insulin analogue LYSPRO in comparison with human regular insulin in healthy subjects. Diabetic Medicine, 10(10), 921-924.
[24] Bergenstal, R. M., Rosenstock, J., Bolli, G. B., & Riddle, M. C. (2018). Safety of new basal insulins: an update of clinical trials. Diabetes Care, 41(11), 2461-2468.
[25] Pickup, J. C. (2012). Insulin-pump therapy for type 1 diabetes mellitus. New England Journal of Medicine, 366(17), 1616-1624.
[26] Bekiari, E., Kitsios, K., Thabit, H., & Tauschmann, M. (2018). Artificial pancreas treatment for outpatients with type 1 diabetes: systematic review and meta-analysis. BMJ, 361, k1310.
[27] Garg, S., & Weinzimer, S. A. (2018). Alternative routes of insulin delivery: where are we now?. Diabetes Technology & Therapeutics, 20(S2), S2-139.
[28] Knowler, W. C., Barrett-Connor, E., Fowler, S. E., Hamman, R. F., Lachin, J. M., Walker, E. A., & Nathan, D. M. (2002). Reduction in the incidence of type 2 diabetes with lifestyle intervention or metformin. New England Journal of Medicine, 346(6), 393-403.
[29] Davis, A. (2018). The Mediterranean diet and type 2 diabetes. Diabetes Spectrum, 31(2), 123-128.
[30] Hawley, J. A., & Lessard, S. J. (2008). Exercise training-induced improvements in insulin action. Acta Physiologica, 192(1), 129-137.
[31] Knutson, K. L., Van Cauter, E., Rathouz, P. J., DeLeire, T., Molenda, A. L., & Niemcryk, M. A. (2007). Association between sleep and blood glucose in a population-based sample of US adults. American Journal of Epidemiology, 166(9), 1068-1074.
[32] Anderson, J. G., Taylor, A. G., Narayan, P., & Floyd, L. D. (2011). Impact of mindfulness meditation on glucose regulation and cardiovascular risk factors in type 2 diabetes: a pilot study. Psychosomatic Medicine, 73(7), 585-592.
[33] Cani, P. D. (2018). Gut microbiota–at the heart of everything?. Nature Reviews Gastroenterology & Hepatology, 14(1), 4-5.
Insulin in the brain? So, are we talking about a future where Alzheimer’s patients get insulin nasal sprays instead of Sudoku? Should we start investing in Big Pharma or Big Tissue? Inquiring minds need to know.