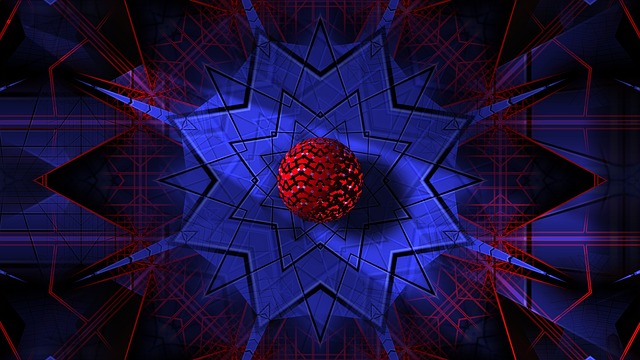
Abstract
This research report provides a comprehensive overview of the rapidly evolving field of advanced materials, focusing on recent advancements in synthesis, characterization techniques, and their applications across diverse sectors. Beyond generative AI-driven discovery, this report explores the broader landscape of materials science, encompassing novel synthesis methodologies, advanced characterization tools that provide deeper insights into material properties, and the application of these materials in fields such as energy storage, biomedical engineering, electronics, and sustainable technologies. The report also addresses challenges related to scalability, cost-effectiveness, and long-term stability of advanced materials, alongside a discussion of future trends and research directions that will shape the field in the coming decades.
Many thanks to our sponsor Esdebe who helped us prepare this research report.
1. Introduction
The field of materials science and engineering is pivotal to technological advancement and societal progress. From the earliest utilization of stone and bronze to the sophisticated semiconductors and polymers of today, materials have consistently shaped our world. The relentless pursuit of materials with enhanced properties, novel functionalities, and sustainable attributes drives ongoing research efforts. This report aims to provide a comprehensive overview of the current state of advanced materials, encompassing synthesis methodologies, characterization techniques, and a broad range of applications. Unlike other reports that may focus primarily on AI-driven discovery, this document examines the entire spectrum of advanced materials research, acknowledging the critical roles of traditional experimental techniques, computational modeling, and innovative processing methods in the development of next-generation materials.
Many thanks to our sponsor Esdebe who helped us prepare this research report.
2. Novel Synthesis Methodologies
The creation of advanced materials often hinges on the development of innovative synthesis techniques that allow for precise control over composition, structure, and morphology. Traditional methods, while valuable, often face limitations in achieving desired material properties or scalability. This section explores several novel synthesis methodologies that are revolutionizing materials fabrication.
2.1. Self-Assembly
Self-assembly is a bottom-up approach where individual building blocks spontaneously organize into ordered structures driven by thermodynamic principles. This method offers precise control over nanoscale architecture and is particularly attractive for creating complex hierarchical materials. Examples include the self-assembly of block copolymers into micelles or lamellae, the formation of colloidal crystals, and the self-organization of DNA into functional nanostructures [1, 2]. The appeal lies in its ability to create intricate patterns and structures without the need for complex lithography or external manipulation. However, challenges remain in controlling the kinetics of self-assembly and ensuring the long-range order of the resulting structures.
2.2. Additive Manufacturing (3D Printing)
Additive manufacturing (AM), commonly known as 3D printing, has emerged as a transformative technology for materials processing. AM techniques, such as selective laser melting (SLM), fused deposition modeling (FDM), and stereolithography (SLA), enable the fabrication of complex geometries with unprecedented design freedom [3]. AM allows for the creation of customized materials with tailored properties, including graded compositions and spatially varying microstructures. The use of AM extends to a wide range of materials, including polymers, metals, ceramics, and composites. Despite its advantages, AM faces challenges related to material porosity, surface finish, and the development of high-throughput processes for mass production.
2.3. Chemical Vapor Deposition (CVD)
CVD is a widely used technique for depositing thin films and coatings with precise control over thickness and composition. In CVD, gaseous precursors react on a heated substrate, forming a solid material. Recent advances in CVD include the development of atomic layer deposition (ALD), which enables the deposition of ultrathin films with atomic-level precision [4]. Plasma-enhanced CVD (PECVD) is another variant that utilizes plasma to lower the deposition temperature, allowing for the fabrication of materials on temperature-sensitive substrates. CVD is crucial for the fabrication of semiconductors, protective coatings, and catalysts. However, the high cost of precursors and the potential for toxic byproducts remain challenges for widespread adoption.
2.4. Solution-Based Synthesis
Solution-based synthesis offers a versatile and cost-effective approach for producing nanomaterials and thin films. This method involves the precipitation of materials from a solution containing dissolved precursors. Hydrothermal synthesis, sol-gel processing, and co-precipitation are common solution-based techniques. These methods allow for control over particle size, shape, and composition by adjusting the reaction conditions, such as temperature, pH, and precursor concentration [5]. Solution-based synthesis is particularly well-suited for the production of nanoparticles, nanowires, and thin films for various applications, including catalysis, energy storage, and biomedical engineering. Scalability and control over particle uniformity are ongoing challenges.
Many thanks to our sponsor Esdebe who helped us prepare this research report.
3. Advanced Characterization Techniques
The advancement of materials science relies heavily on characterization techniques that provide detailed information about the structure, composition, and properties of materials. This section highlights some of the key advanced characterization techniques used in materials research.
3.1. Transmission Electron Microscopy (TEM)
TEM is a powerful technique that allows for the visualization of materials at the atomic level. By transmitting a beam of electrons through a thin sample, TEM can reveal the crystallographic structure, defects, and interfaces of materials with sub-nanometer resolution [6]. High-resolution TEM (HRTEM) can directly image the atomic lattice, providing valuable insights into the material’s structure. Scanning TEM (STEM) offers additional capabilities, such as elemental mapping and electron energy-loss spectroscopy (EELS), enabling the determination of the chemical composition and electronic structure of materials. Sample preparation can be laborious and requires specialized equipment. The interpretation of complex micrographs also requires significant expertise.
3.2. X-ray Diffraction (XRD)
XRD is a widely used technique for determining the crystal structure and phase composition of materials. By analyzing the diffraction pattern of X-rays scattered by a crystalline sample, XRD can identify the lattice parameters, crystallite size, and preferred orientation of the material [7]. XRD is a non-destructive technique that can be used to characterize a wide range of materials, including powders, thin films, and bulk samples. Advances in XRD include the development of high-resolution and synchrotron-based techniques, which provide more detailed structural information. However, XRD is less sensitive to amorphous materials and minor phases.
3.3. Atomic Force Microscopy (AFM)
AFM is a versatile technique for imaging the surface topography of materials with nanometer resolution. AFM uses a sharp tip mounted on a cantilever to scan the surface of a sample [8]. By measuring the deflection of the cantilever, AFM can create a three-dimensional map of the surface. AFM can be operated in various modes, including contact mode, tapping mode, and non-contact mode, allowing for the characterization of a wide range of materials, including soft biological samples. Beyond imaging, AFM can also be used for measuring the mechanical properties of materials, such as elasticity and adhesion. The technique can be slow and sensitive to vibrations and environmental noise.
3.4. Spectroscopy Techniques (XPS, AES, SIMS)
Spectroscopic techniques provide valuable information about the elemental composition, chemical state, and electronic structure of materials. X-ray photoelectron spectroscopy (XPS) measures the binding energies of core-level electrons, providing information about the elemental composition and chemical bonding of the material surface [9]. Auger electron spectroscopy (AES) is another surface-sensitive technique that analyzes the energy of emitted Auger electrons to determine the elemental composition. Secondary ion mass spectrometry (SIMS) measures the mass-to-charge ratio of ions sputtered from the sample surface, providing information about the elemental composition and depth profile of the material [10]. These techniques are essential for characterizing the surface chemistry and electronic properties of materials, but they often require ultra-high vacuum conditions and specialized expertise.
Many thanks to our sponsor Esdebe who helped us prepare this research report.
4. Applications of Advanced Materials
The development of advanced materials is driven by the need for materials with enhanced properties and novel functionalities across various applications. This section highlights some of the key applications of advanced materials.
4.1. Energy Storage
Advanced materials play a critical role in energy storage technologies, such as batteries, supercapacitors, and fuel cells. Lithium-ion batteries, for example, rely on advanced electrode materials with high energy density, high power density, and long cycle life [11]. Nanomaterials, such as carbon nanotubes, graphene, and metal oxides, are being explored as electrode materials to improve the performance of batteries. Supercapacitors utilize materials with high surface area and excellent electrical conductivity, such as activated carbon and conducting polymers, to store energy electrostatically [12]. Fuel cells utilize catalysts made of noble metals or transition metal oxides to facilitate the electrochemical reactions that convert chemical energy into electrical energy [13]. Improving the efficiency, stability, and cost-effectiveness of these materials remains a major challenge.
4.2. Biomedical Engineering
Advanced materials are revolutionizing biomedical engineering, with applications ranging from drug delivery to tissue engineering and medical implants. Biocompatible materials, such as titanium alloys, polymers, and ceramics, are used in orthopedic and dental implants to replace damaged tissues [14]. Nanomaterials, such as liposomes, nanoparticles, and nanofibers, are being explored as drug delivery vehicles to target specific cells or tissues and improve the efficacy of drug treatments [15]. Scaffolds made of biodegradable polymers or extracellular matrix materials are used in tissue engineering to promote cell growth and tissue regeneration [16]. Ensuring biocompatibility, minimizing immune response, and achieving long-term stability are critical considerations in biomedical applications.
4.3. Electronics
Advanced materials are essential for the development of high-performance electronic devices, such as transistors, sensors, and displays. Semiconductors, such as silicon, gallium arsenide, and indium gallium nitride, are the building blocks of transistors and integrated circuits [17]. Two-dimensional materials, such as graphene, molybdenum disulfide, and tungsten diselenide, are being explored for their unique electronic properties and potential applications in flexible electronics [18]. Transparent conducting oxides, such as indium tin oxide (ITO), are used as electrodes in displays and solar cells [19]. The demand for faster, smaller, and more energy-efficient electronic devices drives the ongoing search for new and improved materials.
4.4. Sustainable Technologies
Advanced materials are crucial for developing sustainable technologies that address environmental challenges, such as climate change and pollution. Solar cells rely on materials that can efficiently convert sunlight into electricity [20]. Wind turbines utilize lightweight and strong composite materials to capture wind energy [21]. Catalysts are used to remove pollutants from exhaust gases and industrial waste streams [22]. Membranes made of polymers or ceramics are used for water purification and desalination [23]. The development of sustainable materials that are abundant, non-toxic, and recyclable is essential for creating a more environmentally friendly future. There is also the challenge of the embodied energy in production of these materials and their long term disposal.
Many thanks to our sponsor Esdebe who helped us prepare this research report.
5. Challenges and Future Directions
Despite the significant progress in the field of advanced materials, several challenges remain that need to be addressed to realize the full potential of these materials. One major challenge is the scalability of synthesis methods. Many advanced materials are synthesized using techniques that are not easily scalable to mass production, limiting their widespread adoption. Another challenge is the cost of advanced materials. The synthesis and processing of these materials often require expensive precursors and equipment, making them unaffordable for many applications. The long-term stability and durability of advanced materials are also a concern. Many materials degrade over time due to environmental factors, such as temperature, humidity, and UV radiation, limiting their lifespan. Finally, the environmental impact of advanced materials needs to be carefully considered. The synthesis, processing, and disposal of these materials can generate hazardous waste and contribute to pollution. Addressing these challenges will require interdisciplinary collaborations between materials scientists, engineers, chemists, and environmental scientists.
Future research directions in advanced materials include the development of new synthesis methods that are scalable, cost-effective, and environmentally friendly. This includes exploring new materials informatics, which can help identify promising new materials and predict their properties. Developing novel characterization techniques that provide more detailed information about the structure, composition, and properties of materials is also crucial. This includes the development of in-situ characterization techniques that can monitor materials in real-time during synthesis and operation. Furthermore, research is needed to improve the long-term stability and durability of advanced materials, as well as to minimize their environmental impact. By addressing these challenges and pursuing these research directions, the field of advanced materials can continue to drive technological innovation and contribute to a more sustainable future.
Many thanks to our sponsor Esdebe who helped us prepare this research report.
6. Ethical Considerations
The rapid advancement in materials science, particularly with the integration of AI, brings forth important ethical considerations. The potential for designing materials with enhanced properties raises concerns about dual-use applications, where the same material could be used for beneficial purposes or for harmful ones, such as advanced weaponry or surveillance technologies. The environmental impact of new materials is another key concern. While many advanced materials aim to promote sustainability, their production and disposal can have significant environmental consequences, including pollution and resource depletion. Ensuring responsible innovation requires careful consideration of the potential risks and benefits of new materials, as well as the development of ethical guidelines and regulations to govern their development and use.
Many thanks to our sponsor Esdebe who helped us prepare this research report.
7. Conclusion
The field of advanced materials is a dynamic and rapidly evolving field that holds immense promise for addressing some of the world’s most pressing challenges. From energy storage to biomedical engineering to sustainable technologies, advanced materials are enabling new innovations and improving the quality of life. The development of novel synthesis methods, advanced characterization techniques, and interdisciplinary collaborations are driving the field forward. While challenges remain, the future of advanced materials is bright, and continued research and development will undoubtedly lead to even more groundbreaking discoveries in the years to come.
Many thanks to our sponsor Esdebe who helped us prepare this research report.
References
[1] Whitesides, G. M., & Grzybowski, B. (2002). Self-assembly at all scales. Science, 295(5564), 2418-2421.
[2] Berger, R., de Villeneuve, C. H., Preece, J. A., Li, Z., Beckmann, R., Bohlen, H., … & Ghadiri, M. R. (2000). Peptide Nanotubes. Journal of the American Chemical Society, 122(44), 10735-10741.
[3] Gibson, I., Rosen, D. W., & Stucker, B. (2014). Additive manufacturing technologies: 3D printing, rapid prototyping, and direct digital manufacturing. Springer.
[4] George, S. M. (2010). Atomic layer deposition: an overview. Chemical Reviews, 110(1), 111-131.
[5] Caruso, F. (2000). Hollow capsule processing through a templating approach. Chemical Engineering Science, 55(22), 5035-5051.
[6] Williams, D. B., & Carter, C. B. (2009). Transmission electron microscopy: a textbook for materials science. Springer.
[7] Cullity, B. D., & Stock, S. R. (2001). Elements of X-ray diffraction. Prentice Hall.
[8] Bhushan, B. (2017). Introduction to tribology. John Wiley & Sons.
[9] Briggs, D., & Seah, M. P. (Eds.). (1996). Practical surface analysis: Auger and X-ray photoelectron spectroscopy. John Wiley & Sons.
[10] Benninghoven, A., Rüdenauer, F. G., & Werner, H. W. (1987). Secondary ion mass spectrometry: basic concepts, instrumental aspects, applications and trends. John Wiley & Sons.
[11] Tarascon, J. M., & Armand, M. (2001). Issues and challenges facing rechargeable lithium batteries. Nature, 414(6861), 359-367.
[12] Conway, B. E. (1999). Electrochemical Supercapacitors: Scientific Fundamentals and Technological Applications. Kluwer Academic/Plenum Publishers.
[13] O’Hayre, R., Cha, S. W., Colella, W., & Prinz, F. B. (2016). Fuel cell fundamentals. John Wiley & Sons.
[14] Ratner, B. D., Hoffman, A. S., Schoen, F. J., & Lemons, J. E. (2013). Biomaterials science: an introduction to materials in medicine. Academic Press.
[15] Ferrari, M. (2005). Cancer nanotechnology: opportunities and challenges. Nature Reviews Cancer, 5(3), 161-171.
[16] Langer, R., & Tirrell, D. A. (2004). Designing materials for biology and medicine. Nature, 428(6982), 487-492.
[17] Sze, S. M., & Ng, K. K. (2006). Physics of semiconductor devices. John Wiley & Sons.
[18] Novoselov, K. S., Geim, A. K., Morozov, S. V., Jiang, D., Zhang, Y., Dubonos, S. V., … & Firsov, A. A. (2004). Electric field effect in atomically thin carbon films. Science, 306(5696), 666-669.
[19] Minami, T. (2008). Transparent conducting oxides for transparent electrodes. Semiconductor Science and Technology, 20(4), S35.
[20] Green, M. A. (2003). Third generation photovoltaics: advanced solar energy conversion. Springer.
[21] Manwell, J. F., McGowan, J. G., & Rogers, A. L. (2009). Wind energy explained: theory, design and application. John Wiley & Sons.
[22] Ertl, G., Knözinger, H., & Schüth, F. (2008). Handbook of heterogeneous catalysis. John Wiley & Sons.
[23] Baker, R. W. (2012). Membrane technology and applications. John Wiley & Sons.
The report mentions the challenge of scalability in advanced material synthesis. Could innovations in continuous flow reactors offer a viable path toward more scalable and cost-effective production, particularly for nanomaterials?