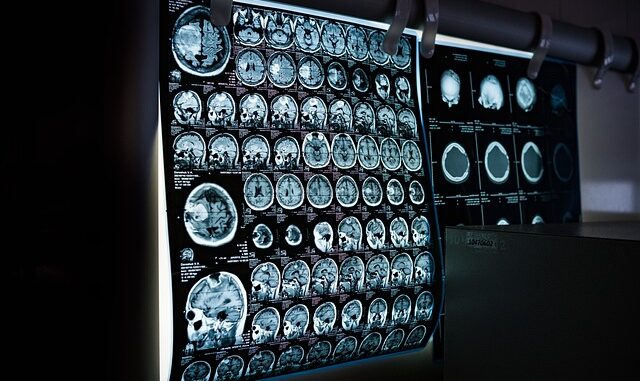
Advancements and Challenges in Modern Fluoroscopy: A Comprehensive Review
Many thanks to our sponsor Esdebe who helped us prepare this research report.
Abstract
Fluoroscopy, a real-time X-ray imaging technique, remains a cornerstone of modern medical practice, offering dynamic visualization of anatomical structures and physiological processes. This research report provides a comprehensive overview of fluoroscopy, examining its fundamental principles, technological advancements, diverse clinical applications, radiation safety considerations, and future directions. We delve into the physics underlying fluoroscopic imaging, exploring the evolution of image intensifiers, flat-panel detectors, and advanced image processing algorithms. The report details the use of fluoroscopy in various medical specialties, including interventional radiology, cardiology, orthopedics, and gastroenterology, highlighting the specific advantages and challenges in each field. A critical analysis of radiation exposure levels associated with fluoroscopic procedures is presented, along with a review of current safety protocols and strategies for dose reduction. Furthermore, we discuss emerging trends and future prospects for fluoroscopy, such as cone-beam computed tomography (CBCT), spectral imaging, and artificial intelligence (AI)-driven image enhancement and automation. This review aims to provide a valuable resource for clinicians, researchers, and engineers working to optimize the clinical utility, safety, and efficiency of fluoroscopic imaging.
Many thanks to our sponsor Esdebe who helped us prepare this research report.
1. Introduction
Fluoroscopy, derived from the Greek words “fluor” (flowing) and “scopy” (viewing), has revolutionized medical imaging since its inception in the late 19th century. Unlike static radiography, fluoroscopy provides dynamic, real-time visualization of anatomical structures and physiological processes, enabling clinicians to guide minimally invasive procedures, diagnose functional abnormalities, and monitor therapeutic interventions. The fundamental principle of fluoroscopy involves continuously irradiating a patient with X-rays and converting the transmitted radiation into a visible image displayed on a monitor. While the basic concept has remained consistent, significant advancements in technology have dramatically improved image quality, reduced radiation dose, and expanded the range of clinical applications.
Initially, fluoroscopic imaging relied on direct viewing of the X-ray beam through a fluorescent screen. This method, however, was limited by low image brightness, poor contrast, and significant radiation exposure to both the patient and the operator. The introduction of image intensifiers in the 1950s marked a significant breakthrough, amplifying the X-ray signal and allowing for indirect viewing of the image on a television monitor. This innovation not only improved image quality but also significantly reduced radiation exposure.
In recent decades, further advancements in detector technology, digital image processing, and computer hardware have led to the development of modern digital fluoroscopy systems. Flat-panel detectors (FPDs), which offer superior spatial resolution, contrast resolution, and dynamic range compared to image intensifiers, have become increasingly prevalent. Advanced image processing algorithms, such as noise reduction, edge enhancement, and motion artifact correction, further enhance image quality and improve diagnostic accuracy. The integration of fluoroscopy with other imaging modalities, such as computed tomography (CT) and magnetic resonance imaging (MRI), has also expanded its capabilities, enabling more precise and targeted interventions.
Despite its numerous advantages, fluoroscopy also presents challenges, primarily related to radiation exposure. The prolonged imaging times and relatively high radiation doses associated with fluoroscopic procedures raise concerns about potential long-term health risks, including cancer. Therefore, it is crucial to optimize imaging protocols, implement radiation safety measures, and explore dose reduction strategies to minimize the risks to both patients and medical personnel. This review will delve into these topics in detail.
Many thanks to our sponsor Esdebe who helped us prepare this research report.
2. Principles of Fluoroscopic Imaging
Fluoroscopy utilizes X-rays to create real-time images of the body’s internal structures. The process involves several key steps:
2.1 X-ray Generation
The fluoroscopic system employs an X-ray tube to generate X-rays. The X-ray tube consists of a cathode (a heated filament that emits electrons) and an anode (a rotating target made of tungsten or other high-atomic-number material). When a high voltage is applied between the cathode and the anode, electrons are accelerated towards the anode. Upon impact, these electrons interact with the anode material, producing X-rays through bremsstrahlung and characteristic radiation. The intensity and energy of the X-ray beam can be controlled by adjusting the tube current (mA) and the tube voltage (kV), respectively.
2.2 X-ray Attenuation
As the X-ray beam passes through the patient’s body, it is attenuated (absorbed or scattered) by different tissues depending on their density and atomic number. Dense tissues, such as bone, attenuate more X-rays than less dense tissues, such as soft tissues. This differential attenuation creates a spatial pattern of X-ray intensity that carries information about the internal anatomy.
2.3 Image Intensification/Detection
The attenuated X-ray beam is then detected by either an image intensifier or a flat-panel detector. In an image intensifier, the X-rays are first converted into light by an input phosphor screen (typically made of cesium iodide). The light is then focused onto a photocathode, which emits electrons via the photoelectric effect. These electrons are accelerated and focused by electrostatic lenses towards an output phosphor screen, where they are converted back into light. The output phosphor screen is much smaller than the input phosphor screen, resulting in a significant amplification of the light signal and a brighter image. This amplified image is then captured by a video camera and displayed on a monitor.
Flat-panel detectors, on the other hand, directly convert X-rays into electrical signals. There are two main types of FPDs: indirect and direct. Indirect FPDs use a scintillator layer (e.g., cesium iodide or gadolinium oxysulfide) to convert X-rays into light, which is then detected by an array of photodiodes. Direct FPDs use a semiconductor layer (e.g., amorphous selenium) to directly convert X-rays into electrical charge, which is then read out by an array of thin-film transistors (TFTs).
FPDs offer several advantages over image intensifiers, including higher spatial resolution, better contrast resolution, wider dynamic range, and lower geometric distortion. They also eliminate the need for vacuum tubes and are less susceptible to magnetic interference.
2.4 Image Processing and Display
The electrical signals from the FPD or the video signal from the image intensifier are processed by a computer to enhance image quality and optimize visualization. Image processing techniques include noise reduction, edge enhancement, contrast adjustment, and motion artifact correction. The processed image is then displayed on a high-resolution monitor for viewing by the clinician.
Many thanks to our sponsor Esdebe who helped us prepare this research report.
3. Technological Advancements in Fluoroscopy
Fluoroscopy technology has undergone significant advancements in recent years, leading to improved image quality, reduced radiation exposure, and expanded clinical applications.
3.1 Flat-Panel Detectors (FPDs)
The transition from image intensifiers to FPDs has been a major advancement in fluoroscopy. FPDs offer several advantages, including:
- Higher spatial resolution: FPDs have smaller pixel sizes, resulting in sharper images and improved visualization of fine details.
- Better contrast resolution: FPDs have a wider dynamic range, allowing for better differentiation between tissues with subtle differences in density.
- Lower geometric distortion: FPDs produce images with less distortion compared to image intensifiers, leading to more accurate measurements and improved guidance of interventional procedures.
- Reduced radiation dose: FPDs are more efficient at converting X-rays into electrical signals, allowing for lower radiation doses to achieve the same image quality.
3.2 Digital Subtraction Angiography (DSA)
DSA is a technique used to visualize blood vessels by subtracting pre-contrast images from post-contrast images. This eliminates the obscuring effects of bone and soft tissue, allowing for clearer visualization of the vasculature. DSA is widely used in interventional radiology for diagnosing and treating vascular diseases.
3.3 Cone-Beam Computed Tomography (CBCT)
CBCT is a three-dimensional imaging technique that uses a cone-shaped X-ray beam to acquire volumetric data. CBCT provides higher spatial resolution and better soft tissue contrast compared to conventional CT, making it useful for a variety of clinical applications, including dental imaging, orthopedic imaging, and interventional radiology.
3.4 Spectral Imaging (Dual-Energy Imaging)
Spectral imaging, also known as dual-energy imaging, uses two different X-ray energy levels to differentiate between tissues based on their attenuation characteristics. This technique can be used to improve contrast resolution, reduce metal artifacts, and identify specific materials within the body.
3.5 Artificial Intelligence (AI) and Machine Learning (ML)
AI and ML are increasingly being used in fluoroscopy to improve image quality, reduce radiation dose, and automate tasks. AI algorithms can be used for:
- Image enhancement: AI algorithms can be trained to remove noise, enhance edges, and improve contrast in fluoroscopic images.
- Dose optimization: AI algorithms can be used to optimize imaging parameters and reduce radiation dose while maintaining image quality.
- Automated image analysis: AI algorithms can be used to automatically detect and quantify anatomical structures, such as blood vessels or fractures.
- Workflow automation: AI can automate various tasks, such as image registration, segmentation, and reporting, thereby improving workflow efficiency.
Many thanks to our sponsor Esdebe who helped us prepare this research report.
4. Clinical Applications of Fluoroscopy
Fluoroscopy is used in a wide range of medical specialties for diagnosis, treatment, and guidance of interventional procedures.
4.1 Interventional Radiology
Fluoroscopy is an indispensable tool in interventional radiology, enabling minimally invasive procedures such as:
- Angiography and angioplasty: Visualization and treatment of blocked or narrowed blood vessels.
- Embolization: Blocking blood vessels to stop bleeding or treat tumors.
- Stent placement: Inserting stents to keep blood vessels open.
- Biopsy: Obtaining tissue samples for diagnosis.
- Drainage: Draining fluid collections, such as abscesses or hematomas.
- Vertebroplasty and kyphoplasty: Stabilizing fractured vertebrae.
4.2 Cardiology
In cardiology, fluoroscopy is used for:
- Coronary angiography and angioplasty: Visualization and treatment of blocked or narrowed coronary arteries.
- Pacemaker and defibrillator implantation: Guiding the placement of cardiac devices.
- Electrophysiology studies: Mapping and ablating abnormal heart rhythms.
4.3 Orthopedics
Fluoroscopy is used in orthopedics for:
- Fracture reduction and fixation: Guiding the placement of screws, plates, and other implants.
- Joint injections: Injecting medications into joints to relieve pain and inflammation.
- Spinal procedures: Guiding the placement of needles and implants for spinal surgery.
4.4 Gastroenterology
In gastroenterology, fluoroscopy is used for:
- Barium studies: Visualizing the esophagus, stomach, and small intestine to diagnose abnormalities such as ulcers, tumors, and strictures.
- Endoscopic retrograde cholangiopancreatography (ERCP): Visualizing the bile ducts and pancreatic ducts to diagnose and treat conditions such as gallstones and pancreatic cancer.
- Gastrostomy tube placement: Guiding the placement of feeding tubes into the stomach.
4.5 Urology
Fluoroscopy assists with a number of urological procedures including:
* Cystography and voiding cystourethrography (VCUG): Used to evaluate the bladder and urethra for abnormalities such as reflux and strictures.
* Ureteral stent placement: Used to relieve urinary obstruction.
* Percutaneous nephrolithotomy (PCNL): A minimally invasive procedure to remove kidney stones.
4.6 Pain Management
As highlighted in the initial context, fluoroscopy is frequently employed in pain management, particularly for procedures like Epidural Steroid Injections (ESIs), facet joint injections, and nerve blocks. It provides real-time visualization to ensure accurate needle placement and medication delivery, maximizing therapeutic efficacy while minimizing the risk of complications. This is crucial for precise targeting of inflamed or compressed nerves, allowing for effective pain relief.
Many thanks to our sponsor Esdebe who helped us prepare this research report.
5. Radiation Safety Considerations
Radiation exposure is a significant concern in fluoroscopy due to the prolonged imaging times and relatively high radiation doses associated with the procedures. It is crucial to implement radiation safety measures to minimize the risks to both patients and medical personnel.
5.1 Radiation Dose Measurement and Units
- Absorbed dose: The amount of energy deposited per unit mass of tissue, measured in Gray (Gy).
- Equivalent dose: The absorbed dose weighted by a radiation weighting factor to account for the relative biological effectiveness of different types of radiation, measured in Sievert (Sv).
- Effective dose: The equivalent dose weighted by tissue weighting factors to account for the different radiosensitivities of different organs and tissues, measured in Sievert (Sv).
5.2 Factors Affecting Radiation Dose
Several factors influence the radiation dose received by patients and medical personnel during fluoroscopic procedures:
- Imaging parameters: Tube voltage (kV), tube current (mA), pulse rate, and imaging time.
- Patient size and body composition: Larger patients and patients with denser tissues require higher radiation doses to achieve adequate image quality.
- Imaging technique: The use of magnification, fluoroscopy time, and number of acquisitions.
- Equipment settings: Automatic brightness control (ABC) and dose reduction features.
- Operator experience: Skilled operators can often perform procedures more quickly and efficiently, reducing radiation exposure.
5.3 Radiation Protection Principles
The three basic principles of radiation protection are:
- Justification: Any radiation exposure must be justified by its benefits.
- Optimization: Radiation doses should be kept as low as reasonably achievable (ALARA), taking into account economic and social factors.
- Limitation: Radiation doses to individuals should not exceed established dose limits.
5.4 Radiation Protection Measures
Several measures can be taken to reduce radiation exposure during fluoroscopic procedures:
- Proper collimation: Restricting the X-ray beam to the area of interest reduces the amount of tissue irradiated.
- Shielding: Using lead aprons, thyroid shields, and lead glasses to protect the body from radiation.
- Distance: Increasing the distance from the X-ray source reduces radiation exposure due to the inverse square law.
- Pulse fluoroscopy: Using pulsed X-rays instead of continuous X-rays reduces radiation dose by allowing time for the detector to reset and reducing the amount of radiation emitted.
- Dose reduction techniques: Using low-dose protocols, virtual collimation, and iterative reconstruction algorithms to reduce radiation dose while maintaining image quality.
- Operator training and education: Ensuring that medical personnel are properly trained in radiation safety principles and techniques.
- Regular equipment maintenance and calibration: Maintaining equipment in good working order ensures that it is operating within specified parameters and that radiation output is accurate.
- Dose monitoring: Using personal dosimeters to monitor radiation exposure to medical personnel.
- Lead Barriers: Permanent or portable lead barriers should be used to shield personnel from scatter radiation.
5.5 Regulatory Guidelines and Dose Limits
Various regulatory bodies, such as the International Commission on Radiological Protection (ICRP) and the National Council on Radiation Protection and Measurements (NCRP), have established dose limits for occupational and public exposure to radiation. It is important to comply with these guidelines to ensure the safety of patients and medical personnel.
Many thanks to our sponsor Esdebe who helped us prepare this research report.
6. Future Directions
Fluoroscopy continues to evolve, with ongoing research and development focused on improving image quality, reducing radiation dose, and expanding clinical applications.
6.1 Advanced Detector Technology
Research is underway to develop new detector materials and designs that offer even higher spatial resolution, better contrast resolution, and lower radiation dose compared to current FPDs. For example, photon-counting detectors, which directly detect individual X-ray photons, have the potential to significantly reduce radiation dose and improve image quality.
6.2 Spectral Imaging and Material Decomposition
Spectral imaging techniques are being further developed to allow for more accurate material decomposition and improved visualization of specific tissues and materials within the body. This could be useful for identifying calcifications, differentiating between different types of soft tissues, and detecting metal artifacts.
6.3 Artificial Intelligence and Deep Learning
AI and deep learning are expected to play an increasingly important role in fluoroscopy. AI algorithms can be used to automate tasks, improve image quality, reduce radiation dose, and assist with diagnosis and treatment planning. For example, AI algorithms could be used to automatically detect and quantify anatomical structures, predict the outcome of interventional procedures, and optimize imaging parameters for individual patients.
6.4 Integration with Other Imaging Modalities
The integration of fluoroscopy with other imaging modalities, such as CT and MRI, is expected to become more common. This will allow for more comprehensive and personalized imaging, enabling clinicians to make more informed decisions about diagnosis and treatment. For example, fluoroscopy could be used to guide the placement of needles or catheters during CT-guided or MRI-guided interventions.
6.5 Enhanced Visualization Techniques
Novel visualization techniques, such as augmented reality (AR) and virtual reality (VR), are being explored to enhance the visualization of fluoroscopic images. AR and VR could be used to overlay fluoroscopic images onto the patient’s body, providing clinicians with a more intuitive and immersive view of the anatomy during interventional procedures.
Many thanks to our sponsor Esdebe who helped us prepare this research report.
7. Conclusion
Fluoroscopy remains an essential imaging modality in modern medicine, providing real-time visualization for a wide range of diagnostic and therapeutic procedures. Technological advancements, such as flat-panel detectors, cone-beam computed tomography, and artificial intelligence, have significantly improved image quality, reduced radiation dose, and expanded the clinical applications of fluoroscopy. However, radiation safety remains a critical concern, and it is essential to implement appropriate radiation protection measures to minimize the risks to both patients and medical personnel. Ongoing research and development are focused on further improving the performance and safety of fluoroscopy, with the goal of providing clinicians with the best possible tools for diagnosis and treatment.
Many thanks to our sponsor Esdebe who helped us prepare this research report.
References
- Bushong, S. C. (2017). Radiologic science for technologists: Physics, biology, and protection. Elsevier Health Sciences.
- Christensen, E. E., Curry, T. S., & Dowdey, J. E. (1990). An introduction to the physics of diagnostic radiology. Lea & Febiger.
- Hendee, W. R., Ritenour, E. R., & Medical Physics Pub. (2002). Medical imaging physics. Wiley-Liss.
- ICRP. (2007). The 2007 Recommendations of the International Commission on Radiological Protection. ICRP Publication 103. Ann. ICRP 37 (2-4).
- Martin, C. J. (2017). Radiation dose and safety in fluoroscopy. British Journal of Radiology, 90(1078), 20161069.
- NCRP. (2004). Structural Shielding Design for Medical X-Ray Imaging Facilities. NCRP Report No. 147. National Council on Radiation Protection and Measurements.
- Seibert, J. A., Morin, R. L. (2011). The standardized exposure index for digital radiography: An opportunity for optimization of radiation dose to the patient. American Association of Physicists in Medicine. 38 (2): 815-826.
- Sodickson, A., Baeyens, P. F., Pereira, P., & Harrow, J. J. (2004). Occupational radiation exposure associated with interventional procedures: a prospective study. Archives of Internal Medicine, 164(19), 2069-2073.
- Tsapaki, V., Kottou, S., & Vano, E. (2015). Patient and staff doses in interventional cardiology. Radiation Protection Dosimetry, 165(1-4), 192-195.
- Vano, E., Miller, D. L., Martin, C. J., Padovani, R., & Ciraj-Bjelac, O. (2009). Occupational radiation doses in interventional radiology: a review. Radiation Protection Dosimetry, 136(4), 272-278.
- Yu, L., Li, H., Leng, S., McCollough, C. H. (2009). Dual-energy CT with added tin filter: physics and clinical applications. American Journal of Roentgenology. 192(6): 1516-1524.
The discussion of AI-driven image enhancement is compelling. Could AI also be leveraged to predict optimal fluoroscopy parameters based on patient-specific factors, further minimizing radiation exposure while maintaining diagnostic quality?
That’s a fantastic point! Exploring AI to predict optimal fluoroscopy parameters based on individual patient characteristics could be a game-changer. Imagine the potential for personalized protocols that significantly reduce radiation exposure while maintaining diagnostic accuracy. It could really tailor the experience. Let’s discuss what patient-specific factors would be most impactful.
Editor: MedTechNews.Uk
Thank you to our Sponsor Esdebe
Given AI’s potential in image enhancement, could we explore its application in real-time artifact reduction during fluoroscopy, specifically for motion or metallic artifacts? What level of computational power would be necessary for such live processing?
That’s a great question! Real-time artifact reduction, particularly for motion and metallic artifacts, is a key area of interest. Exploring the computational power needed is crucial. I’d love to hear more about the specific hardware configurations you think might be most promising for live processing, and what software considerations are required.
Editor: MedTechNews.Uk
Thank you to our Sponsor Esdebe
This comprehensive review highlights crucial advancements. The discussion of AI-driven automation opens exciting possibilities, especially regarding streamlined workflows and reduced operational overhead in busy radiology departments. What are your thoughts on the ethical considerations of increasing automation?
Thanks for your insightful comment! The potential for AI to streamline workflows is definitely exciting. Thinking about the ethical considerations is vital as we move forward. Ensuring fairness, transparency, and accountability in AI-driven systems will be key to responsible implementation across radiology departments. What frameworks should guide AI development and deployment?
Editor: MedTechNews.Uk
Thank you to our Sponsor Esdebe