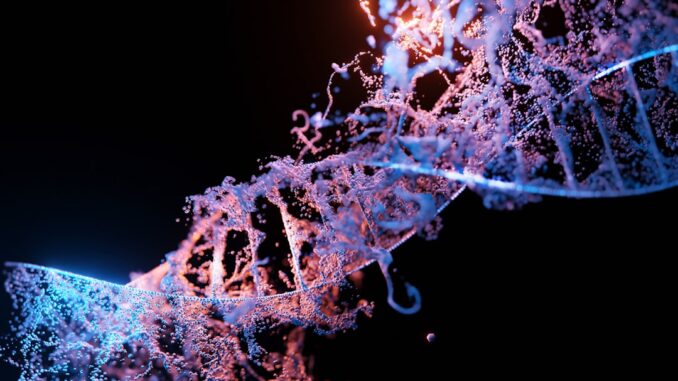
Abstract
Deoxyribonucleic acid (DNA), the blueprint of life, extends far beyond its well-established role in protein-coding. This report delves into the intricate landscape of DNA, encompassing both coding and noncoding regions, their diverse functions, and the implications of mutations within these domains for human health. We examine the complex regulatory networks orchestrated by noncoding DNA, its crucial structural roles, and the burgeoning understanding of how alterations in these regions contribute to various disorders. Furthermore, we address the challenges inherent in studying noncoding DNA, highlighting the limitations of traditional methods and the emergence of cutting-edge technologies, including artificial intelligence (AI)-driven approaches, for unraveling its mysteries. Finally, we explore the broader implications of DNA research, discussing its potential for personalized medicine, targeted therapies, and a deeper comprehension of the fundamental processes that govern life itself. This report aims to provide a comprehensive overview for experts in the field, fostering a deeper appreciation for the multifaceted roles of DNA in the intricate tapestry of life and disease.
Many thanks to our sponsor Esdebe who helped us prepare this research report.
1. Introduction
Deoxyribonucleic acid (DNA) stands as the fundamental molecule of heredity, encoding the genetic instructions that govern the development, function, and reproduction of all known living organisms and many viruses. While the initial focus of genetics centered around the protein-coding regions of DNA, often referred to as genes, it has become increasingly clear that the vast majority of the genome, particularly in complex eukaryotes, is noncoding. This noncoding DNA, initially dismissed as “junk DNA,” is now recognized as playing critical roles in gene regulation, structural organization of the genome, and even evolution itself. This report aims to provide a comprehensive overview of the multifaceted roles of DNA, encompassing both coding and noncoding regions, and to explore the implications of mutations within these domains for human health and disease. Given the sheer complexity and scale of the genome, understanding its function requires a multi-faceted approach, integrating experimental techniques with computational modeling and advanced analytical methods.
The central dogma of molecular biology, while a useful simplification, has been significantly refined over the past few decades. The traditional view of a linear flow of information from DNA to RNA to protein has been challenged by the discovery of regulatory RNAs, complex epigenetic modifications, and intricate feedback loops that dynamically modulate gene expression. This intricate interplay between different genomic elements highlights the need for a holistic understanding of the genome, where both coding and noncoding regions are considered integral components of a complex regulatory network.
The consequences of DNA mutations, particularly in noncoding regions, are increasingly recognized as significant contributors to a wide range of human diseases, including cancer, neurological disorders, and developmental abnormalities. Understanding the mechanisms by which these mutations disrupt normal cellular function is crucial for developing effective diagnostic and therapeutic strategies. This report will delve into the current understanding of these mechanisms, highlighting the challenges and opportunities for future research in this area.
Many thanks to our sponsor Esdebe who helped us prepare this research report.
2. Coding DNA: The Foundation of Protein Synthesis
The protein-coding regions of DNA, typically representing only a small fraction of the genome in complex organisms (e.g., roughly 1-2% in humans), are the blueprints for the synthesis of proteins. These regions are organized into genes, each encoding a specific protein or polypeptide. The process of protein synthesis involves two major steps: transcription and translation.
Transcription is the process by which the DNA sequence of a gene is copied into a messenger RNA (mRNA) molecule. This process is catalyzed by RNA polymerase, which binds to the promoter region of the gene and synthesizes a complementary RNA strand. The mRNA molecule then undergoes processing, including splicing, capping, and polyadenylation, to prepare it for translation.
Translation is the process by which the mRNA sequence is decoded by ribosomes to synthesize a protein. Ribosomes bind to the mRNA molecule and move along it, reading the codons (three-nucleotide sequences) in the mRNA. Each codon specifies a particular amino acid, which is added to the growing polypeptide chain. The process continues until a stop codon is encountered, signaling the end of the protein.
The fidelity of DNA replication and transcription is crucial for maintaining the integrity of the genetic code. However, errors can occur, resulting in mutations. Mutations in coding regions can have a variety of effects, ranging from no effect to complete loss of protein function. These mutations can contribute to a wide range of genetic disorders, including cystic fibrosis, sickle cell anemia, and Huntington’s disease.
Many thanks to our sponsor Esdebe who helped us prepare this research report.
3. Noncoding DNA: Beyond Protein Synthesis
Noncoding DNA encompasses all DNA sequences that do not directly encode proteins. This vast and complex region of the genome has been the subject of intense research in recent years, revealing its critical roles in gene regulation, structural organization of the genome, and evolution. Noncoding DNA can be broadly classified into several categories, including:
- Regulatory sequences: These sequences, such as promoters, enhancers, and silencers, bind transcription factors and other regulatory proteins to control gene expression. They can be located near or far from the genes they regulate, and they often exhibit cell-type-specific activity.
- Introns: These are noncoding sequences located within genes that are transcribed into pre-mRNA but are removed by splicing during mRNA processing. Introns can contain regulatory elements and may play a role in alternative splicing.
- Long noncoding RNAs (lncRNAs): These are RNA molecules longer than 200 nucleotides that do not encode proteins. LncRNAs can regulate gene expression at multiple levels, including transcription, splicing, translation, and chromatin remodeling. They are involved in a wide range of biological processes, including development, immunity, and cancer.
- Short noncoding RNAs: These include microRNAs (miRNAs), small interfering RNAs (siRNAs), and Piwi-interacting RNAs (piRNAs). These RNAs are involved in post-transcriptional gene silencing, often by binding to mRNA molecules and blocking their translation or promoting their degradation.
- Transposable elements: These are DNA sequences that can move from one location to another in the genome. They can contribute to genome instability and can also influence gene expression.
- Structural DNA: This includes centromeres and telomeres, which are essential for chromosome stability and segregation during cell division. Additionally, scaffold/matrix attachment regions (S/MARs) are involved in organizing chromatin structure within the nucleus.
- Pseudogenes: These are nonfunctional copies of genes that have accumulated mutations over time. While typically considered nonfunctional, some pseudogenes have been shown to have regulatory roles.
The functions of noncoding DNA are diverse and complex, and they are still being actively investigated. However, it is clear that noncoding DNA plays a critical role in regulating gene expression, maintaining genome stability, and shaping the evolution of complex organisms. The disruption of these functions through mutations in noncoding regions can have profound consequences for human health.
Many thanks to our sponsor Esdebe who helped us prepare this research report.
4. The Role of Noncoding DNA in Gene Regulation
Gene regulation is a complex process that involves the precise control of gene expression in response to a variety of internal and external signals. Noncoding DNA plays a crucial role in this process, acting as a platform for the binding of transcription factors and other regulatory proteins.
Enhancers and silencers, located near or far from the genes they regulate, control gene transcription. These regions contain binding sites for transcription factors, which can either activate or repress gene expression. These regulatory sequences can function across large genomic distances, sometimes acting on genes located on different chromosomes. The spatial organization of the genome within the nucleus, including chromatin looping and higher-order chromatin structures, plays a crucial role in bringing enhancers and promoters into proximity, facilitating gene regulation.
LncRNAs are emerging as key regulators of gene expression, acting through a variety of mechanisms. They can bind to DNA, RNA, or proteins, and they can influence transcription, splicing, translation, and chromatin remodeling. Some lncRNAs act as scaffolds, bringing together different proteins to form regulatory complexes. Others act as decoys, sequestering transcription factors or other regulatory proteins. Still others act as guides, directing regulatory complexes to specific locations in the genome. The functional diversity of lncRNAs is remarkable, and their roles in gene regulation are only beginning to be understood.
Short noncoding RNAs, such as miRNAs and siRNAs, are also important regulators of gene expression. These RNAs bind to mRNA molecules and block their translation or promote their degradation. MiRNAs are particularly important in development and differentiation, regulating the expression of hundreds of genes. SiRNAs are typically involved in silencing foreign or aberrant RNA molecules, such as those produced by viruses or transposable elements. The ability of these small RNAs to fine-tune gene expression makes them essential components of the cellular regulatory machinery.
Many thanks to our sponsor Esdebe who helped us prepare this research report.
5. Structural Roles of Noncoding DNA
Beyond gene regulation, noncoding DNA plays essential roles in maintaining the structural integrity of the genome and organizing it within the nucleus. Centromeres, composed of repetitive DNA sequences and specialized proteins, are critical for chromosome segregation during cell division. Telomeres, located at the ends of chromosomes, protect them from degradation and prevent them from fusing with other chromosomes. These repetitive sequences are essential for maintaining genome stability.
Scaffold/matrix attachment regions (S/MARs) are DNA sequences that bind to the nuclear matrix, a proteinaceous network that provides structural support to the nucleus. S/MARs are thought to play a role in organizing chromatin structure within the nucleus, creating loops of DNA that are accessible to transcription factors. These regions are crucial for proper gene expression and DNA replication.
The three-dimensional organization of the genome within the nucleus, often referred to as chromatin architecture, is increasingly recognized as an important factor in gene regulation. Noncoding DNA plays a critical role in shaping this architecture, influencing the interactions between different genomic regions and affecting gene expression. Techniques such as chromosome conformation capture (3C) and its derivatives (Hi-C) have provided insights into the spatial organization of the genome, revealing the existence of topologically associating domains (TADs), which are self-interacting genomic regions that are thought to promote local gene regulation.
Many thanks to our sponsor Esdebe who helped us prepare this research report.
6. Mutations in Noncoding DNA and Disease
Mutations in noncoding DNA can have a wide range of effects, depending on the location and nature of the mutation. Mutations in regulatory sequences can alter gene expression, leading to developmental abnormalities, cancer, and other diseases. Mutations in lncRNAs can disrupt their function, affecting gene regulation at multiple levels. Mutations in structural DNA can compromise genome stability, leading to chromosome abnormalities and cancer.
Several specific examples illustrate the role of noncoding DNA mutations in disease:
- Cancer: Mutations in enhancer regions have been implicated in a variety of cancers, leading to the overexpression of oncogenes or the downregulation of tumor suppressor genes. LncRNAs are frequently dysregulated in cancer, and mutations in lncRNA genes can contribute to tumorigenesis. Mutations in telomeres can lead to genomic instability and cancer development.
- Neurological disorders: Mutations in noncoding DNA have been linked to several neurological disorders, including Alzheimer’s disease, Parkinson’s disease, and autism spectrum disorder. These mutations can affect gene expression in the brain, leading to neuronal dysfunction and neurodegeneration.
- Developmental abnormalities: Mutations in regulatory sequences can disrupt developmental processes, leading to congenital defects and other developmental abnormalities. These mutations can affect the expression of genes that are critical for development, such as those involved in cell differentiation and morphogenesis.
- Autoimmune diseases: Genetic variants in non-coding regions have been associated with autoimmune diseases such as rheumatoid arthritis and systemic lupus erythematosus. These variants often influence the expression of immune-related genes, contributing to the dysregulation of the immune system.
It is important to note that the effects of noncoding DNA mutations can be complex and context-dependent. The same mutation may have different effects in different cell types or in different individuals, depending on their genetic background and environmental exposures. This complexity makes it challenging to identify and characterize the functional consequences of noncoding DNA mutations.
Many thanks to our sponsor Esdebe who helped us prepare this research report.
7. Challenges in Studying Noncoding DNA
Studying noncoding DNA presents several significant challenges. First, the sheer size and complexity of noncoding regions make it difficult to identify functionally relevant sequences. Unlike protein-coding genes, which can be readily identified by their open reading frames, noncoding DNA sequences often lack obvious features that distinguish them from the surrounding DNA. Furthermore, the functions of noncoding DNA sequences can be highly context-dependent, making it difficult to predict their effects based on sequence alone.
Second, many noncoding DNA sequences exhibit cell-type-specific activity, meaning that their functions vary depending on the cell type in which they are expressed. This necessitates the use of cell-type-specific assays to study their functions. However, obtaining sufficient amounts of specific cell types for analysis can be challenging, especially for rare or difficult-to-isolate cell populations.
Third, the effects of noncoding DNA mutations can be subtle and difficult to detect. Unlike mutations in protein-coding genes, which can have dramatic effects on protein function, mutations in noncoding DNA may only subtly alter gene expression or chromatin structure. This requires the use of sensitive and quantitative assays to detect these subtle effects.
Finally, the lack of conservation of noncoding DNA sequences across species makes it difficult to use comparative genomics to identify functionally important regions. While protein-coding genes are often highly conserved across species, noncoding DNA sequences tend to be more variable, reflecting their roles in adaptation and evolution. This makes it challenging to extrapolate findings from model organisms to humans.
Many thanks to our sponsor Esdebe who helped us prepare this research report.
8. Current Methods for Analyzing Noncoding DNA
Despite the challenges, significant progress has been made in developing methods for analyzing noncoding DNA. These methods can be broadly classified into experimental approaches and computational approaches.
Experimental approaches:
- Reporter assays: These assays involve inserting a candidate noncoding DNA sequence upstream of a reporter gene and measuring the expression of the reporter gene in different cell types or under different conditions. This allows researchers to identify regulatory sequences that can activate or repress gene expression.
- Chromatin immunoprecipitation (ChIP): This technique involves using antibodies to isolate DNA fragments that are bound to specific proteins, such as transcription factors or histone modifications. This allows researchers to identify the genomic locations where these proteins are bound, providing insights into the regulatory roles of noncoding DNA.
- RNA sequencing (RNA-seq): This technique involves sequencing the RNA molecules in a sample, providing a comprehensive view of gene expression. RNA-seq can be used to identify lncRNAs and other noncoding RNAs, as well as to measure the expression levels of protein-coding genes.
- CRISPR-Cas9 genome editing: This technology allows researchers to precisely edit the genome, including noncoding regions. This can be used to delete, insert, or modify noncoding DNA sequences and to study the effects of these changes on gene expression and cellular function.
- Chromosome conformation capture (3C) and its derivatives (Hi-C, ChIA-PET): These techniques map the three-dimensional organization of the genome within the nucleus, providing insights into the interactions between different genomic regions. These techniques can be used to identify enhancer-promoter interactions and to study the roles of noncoding DNA in shaping chromatin architecture.
Computational approaches:
- Sequence motif analysis: This involves searching for recurring patterns of DNA sequences that are associated with specific functions, such as transcription factor binding sites. This can be used to identify candidate regulatory sequences within noncoding DNA.
- Machine learning: Machine learning algorithms can be trained to predict the functions of noncoding DNA sequences based on their sequence features, epigenetic marks, and other data. This can be used to prioritize candidate noncoding DNA sequences for further experimental investigation.
- Genome-wide association studies (GWAS): GWAS involve scanning the genome for genetic variants that are associated with a particular trait or disease. GWAS can identify noncoding DNA variants that contribute to disease risk.
- Network analysis: This involves constructing networks of interacting genes and noncoding RNAs and analyzing the properties of these networks to identify key regulatory elements. This can be used to understand the complex interactions between different genomic components and their roles in disease.
Many thanks to our sponsor Esdebe who helped us prepare this research report.
9. The Role of AI in Analyzing Noncoding DNA
Artificial intelligence (AI) is rapidly transforming the field of genomics, offering powerful tools for analyzing the vast and complex datasets generated by modern sequencing technologies. AI-based approaches are particularly well-suited for studying noncoding DNA, which presents significant challenges due to its complexity and lack of clear functional signals.
Machine learning algorithms, such as deep neural networks, can be trained to predict the functions of noncoding DNA sequences based on their sequence features, epigenetic marks, and other data. These algorithms can identify subtle patterns that are not easily detected by traditional methods, allowing researchers to predict the regulatory potential of noncoding regions with greater accuracy. For example, deep learning models have been successfully used to predict enhancer activity, transcription factor binding sites, and the effects of noncoding DNA mutations on gene expression. DeepBind and related tools are capable of predicting the binding affinities of proteins to specific DNA sequences, including regulatory regions. This allows researchers to identify potential transcription factor binding sites and understand how variations in DNA sequence might alter protein-DNA interactions.
AI can also be used to integrate different types of genomic data, such as sequence data, epigenetic data, and gene expression data, to build more comprehensive models of gene regulation. This can help to identify the complex interactions between different genomic components and their roles in disease. For instance, AI can integrate ChIP-seq data with RNA-seq data to identify regulatory elements that are actively influencing gene expression. Furthermore, AI algorithms can be used to prioritize candidate noncoding DNA variants for further experimental investigation. By integrating GWAS data with functional genomic data, AI can identify variants that are most likely to be causally related to disease. Programs like CADD (Combined Annotation Dependent Depletion) use machine learning to predict the deleteriousness of genetic variants, including those in non-coding regions, based on a variety of genomic annotations.
However, it is important to acknowledge the limitations of AI-based approaches. These algorithms are only as good as the data on which they are trained, and they can be biased by the training data. Furthermore, AI models are often “black boxes,” meaning that it can be difficult to understand how they make their predictions. This can limit their interpretability and make it challenging to validate their findings. Addressing these limitations will require careful attention to data quality, model validation, and the development of more transparent and interpretable AI algorithms. Despite these challenges, AI has the potential to revolutionize the study of noncoding DNA and to accelerate the discovery of new therapeutic targets for human diseases.
Many thanks to our sponsor Esdebe who helped us prepare this research report.
10. Implications for Personalized Medicine and Targeted Therapies
Understanding the roles of both coding and noncoding DNA in health and disease has profound implications for personalized medicine and the development of targeted therapies. By identifying the specific genetic variations that contribute to disease risk, it may be possible to develop personalized diagnostic and therapeutic strategies that are tailored to the individual patient.
For example, patients with cancer could have their genomes sequenced to identify mutations in noncoding regions that are driving their disease. This information could be used to select targeted therapies that specifically inhibit the activity of these mutated regulatory elements. Similarly, patients with neurological disorders could have their genomes sequenced to identify noncoding DNA mutations that are affecting gene expression in the brain. This information could be used to develop therapies that restore normal gene expression patterns.
The development of CRISPR-Cas9 genome editing technology offers the potential to directly correct disease-causing mutations in noncoding DNA. This could be used to treat a wide range of genetic disorders, including those caused by mutations in regulatory sequences, lncRNAs, or structural DNA. However, it is important to acknowledge the ethical and safety concerns associated with genome editing, and further research is needed to ensure that this technology is used responsibly.
Personalized medicine based on an understanding of coding and noncoding DNA could also lead to more effective prevention strategies. By identifying individuals who are at high risk for developing certain diseases, it may be possible to implement lifestyle changes or preventive therapies that reduce their risk. This could have a significant impact on public health and reduce the burden of disease.
Many thanks to our sponsor Esdebe who helped us prepare this research report.
11. Future Directions and Conclusion
The study of DNA, encompassing both coding and noncoding regions, has undergone a dramatic transformation in recent years. The recognition of the critical roles of noncoding DNA in gene regulation, structural organization of the genome, and disease has opened up new avenues for research and therapeutic development. The ongoing advancements in sequencing technologies, genome editing techniques, and AI-based analytical methods are poised to further accelerate our understanding of the complex interplay between different genomic elements.
Future research efforts should focus on:
- Developing more comprehensive maps of regulatory elements within noncoding DNA: This will require the use of high-throughput experimental techniques and sophisticated computational methods to identify and characterize the functions of enhancers, silencers, and other regulatory sequences.
- Unraveling the mechanisms by which lncRNAs regulate gene expression: This will require the development of new tools and approaches to study the interactions between lncRNAs and other molecules, such as DNA, RNA, and proteins.
- Investigating the roles of noncoding DNA in shaping chromatin architecture: This will require the use of advanced imaging techniques and computational modeling to study the three-dimensional organization of the genome within the nucleus.
- Identifying the specific noncoding DNA mutations that contribute to disease: This will require the use of large-scale genomic studies and functional validation assays to identify and characterize the causal variants that are driving disease.
- Developing targeted therapies that specifically target noncoding DNA elements: This will require the development of new drug discovery strategies and delivery methods to target noncoding DNA sequences with high precision.
The future of DNA research is bright, and the potential for improving human health is immense. By embracing new technologies and fostering interdisciplinary collaborations, we can continue to unravel the mysteries of the genome and unlock its full therapeutic potential. The shift from viewing non-coding DNA as “junk” to recognizing its dynamic roles represents a fundamental advancement in our understanding of biology. Continued exploration of these complex regions will undoubtedly lead to breakthroughs in disease diagnosis, treatment, and prevention.
Many thanks to our sponsor Esdebe who helped us prepare this research report.
References
- ENCODE Project Consortium. (2012). An integrated encyclopedia of DNA elements in the human genome. Nature, 489(7414), 57-74.
- Mattick, J. S., & Amaral, P. P. (2011). RNA regulation: a new genetics?. Nature Reviews Genetics, 12(5), 316-323.
- Djebali, S., Davis, C. A., Merkel, A., Dobin, A., Lassmann, T., Tanzer, A., … & Hubbard, T. J. (2012). Landscape of transcription in human cells. Nature, 489(7414), 101-108.
- Li, W., & Godley, L. A. (2013). The functional importance of noncoding DNA in cancer. Journal of Clinical Oncology, 31(30), 3949-3961.
- Consortium, G. T. E. P. (2017). Genetic effects on gene expression across human tissues. Nature, 550(7675), 204-213.
- Andersson, R., Gebhard, C., Miguel-Escalada, I., Hoof, I., Bornholdt, J., Boyd, M., … & Sandelin, A. (2014). An atlas of active enhancers across human cell types and tissues. Nature, 507(7493), 455-461.
- Rinn, J. L., & Chang, H. Y. (2012). Genome regulation by long noncoding RNAs. Annual Review of Biochemistry, 81, 145-166.
- Esteller, M. (2011). Non-coding RNAs in human disease. Nature Reviews Genetics, 12(12), 861-874.
- Lee, T. I., & Young, R. A. (2013). Transcriptional regulation and its misregulation in disease. Cell, 152(6), 1237-1251.
- Uffelmann, E., Boyle, E. A., Carter, H., Spiliopoulou, A., Livanos, G., Huffman, J. E., … & Magi, R. (2021). Genome-wide association studies. Nature Reviews Methods Primers, 1(1), 1-21.
- Zhou, J., Theesfeld, C. L., Yao, K., Chen, J., Hoglen, B. J., Gonzales, N. M., … & Troyanskaya, O. G. (2015). Deep learning sequence-based ab initio prediction of variant effects on RNA splicing. Nature genetics, 47(9), 1004-1009.
- Alipanahi, B., Delong, A., Weirauch, M. T., & Frey, B. J. (2015). Predicting the sequence specificities of DNA-and RNA-binding proteins by deep learning. Nature biotechnology, 33(8), 831-838.
- Kircher, M., Witten, D. M., Jain, P., O’Roak, B. J., Cooper, G. M., & Shendure, J. (2014). A general framework for estimating the relative pathogenicity of human genetic variants. Nature genetics, 46(3), 310-315.
- Xiong, H. Y., Helmer, A. I., Won, H., & Geschwind, D. H. (2016). Modeling the contribution of noncoding genetic variants to autism risk. American Journal of Human Genetics, 99(2), 324-336.
- Khurana, E., Raghupathy, V., Chen, X., Singhal, V., Nizamuddin, S., Akdemir, K. C., … & Levine, R. L. (2016). Integrative annotation of variants reveals mechanisms underlying human disease. Science, 353(6294), 129-135.
So, if AI is now decoding “junk DNA,” does that mean my car’s check engine light will finally tell me *exactly* what’s wrong, or just offer a vague, AI-generated poem about combustion? Asking for a mechanic… who is also me.
That’s a fantastic analogy! While we’re not *quite* at the point of AI diagnosing car troubles with poetic precision, the advances in understanding noncoding DNA are indeed about deciphering complex systems. Perhaps future AI could analyze car diagnostics data with similar techniques, maybe offering a service manual in verse! Thanks for sparking that thought!
Editor: MedTechNews.Uk
Thank you to our Sponsor Esdebe
Given the challenges in interpreting noncoding DNA mutations, how can we better standardize functional validation assays to improve the reliability and reproducibility of research findings across different labs and datasets?
That’s a crucial question! Standardizing functional validation assays is definitely key. Perhaps collaborative efforts to establish benchmark datasets and share detailed protocols would improve cross-lab consistency. Further development of accessible, user-friendly computational tools could also assist in the accurate interpretation of assay results. What are your thoughts on the most pressing obstacles?
Editor: MedTechNews.Uk
Thank you to our Sponsor Esdebe
The report highlights the growing importance of AI in analyzing noncoding DNA. Could AI’s pattern recognition capabilities be further leveraged to predict novel non-coding RNA structures and their potential functions, possibly accelerating therapeutic target discovery?
That’s an excellent point! Exploring the potential of AI to predict novel non-coding RNA structures is a very exciting area. Combining AI predictions with advanced structural biology techniques could significantly accelerate the identification and validation of new therapeutic targets. It would be great to see more research in this area.
Editor: MedTechNews.Uk
Thank you to our Sponsor Esdebe