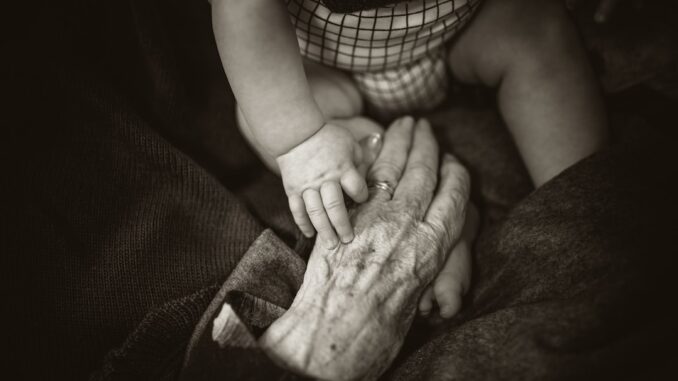
Abstract
Biological age, reflecting an individual’s physiological state rather than chronological age, has emerged as a powerful predictor of healthspan and lifespan. This research report provides a comprehensive overview of biological age, encompassing its multifaceted measurement techniques, intricate determinants, and profound implications for disease risk, with a particular focus on neurodegenerative diseases. We delve into various methodologies for assessing biological age, including epigenetic clocks, transcriptomic signatures, proteomic profiling, metabolomic analysis, and composite biomarker indices. Furthermore, we dissect the complex interplay of genetic, lifestyle, and environmental factors that shape an individual’s biological age trajectory. The report also explores potential interventions aimed at modulating biological aging, such as dietary restriction, exercise, and emerging pharmacological approaches. Finally, we address the ethical considerations surrounding the use of biological age as a predictor of health outcomes and its potential impact on healthcare resource allocation and societal perceptions of aging.
Many thanks to our sponsor Esdebe who helped us prepare this research report.
1. Introduction
Chronological age, while a fundamental demographic variable, often fails to accurately reflect an individual’s underlying health status and susceptibility to age-related diseases. This disparity has fueled the burgeoning field of biological aging research, which seeks to quantify an individual’s physiological age and identify the factors that drive its progression. Biological age, also referred to as physiological age, represents the cumulative impact of genetic predispositions, environmental exposures, and lifestyle choices on an organism’s cellular and molecular integrity. It serves as a more accurate predictor of healthspan, lifespan, and the risk of developing age-related diseases than chronological age alone. The study of biological age has profound implications for understanding the aging process, developing targeted interventions to promote healthy aging, and improving the prediction and prevention of age-related diseases such as cardiovascular disease, cancer, neurodegenerative disorders, and metabolic syndromes.
This report aims to provide a comprehensive review of the current state of biological age research. We will explore the various methods used to measure biological age, delve into the complex determinants that influence its trajectory, examine its relationship with disease risk, and discuss potential interventions to slow down the aging process. Furthermore, we will address the ethical considerations associated with the use of biological age as a predictor of health outcomes.
Many thanks to our sponsor Esdebe who helped us prepare this research report.
2. Measurement of Biological Age
A central challenge in biological age research lies in the accurate and reliable measurement of this complex construct. A single ‘magic bullet’ biomarker that perfectly captures the essence of biological age remains elusive. Instead, researchers have developed a range of methodologies, each with its own strengths and limitations. These can be broadly categorized into epigenetic clocks, transcriptomic signatures, proteomic profiling, metabolomic analysis, and composite biomarker indices.
2.1 Epigenetic Clocks
Epigenetic clocks, particularly those based on DNA methylation patterns, have emerged as highly promising tools for assessing biological age. DNA methylation, a chemical modification of DNA involving the addition of a methyl group to a cytosine base, plays a crucial role in regulating gene expression and maintaining genomic stability. Age-related changes in DNA methylation patterns are highly reproducible and correlate strongly with chronological age, making them ideal candidates for biological age estimation. The development of the Horvath clock, based on DNA methylation levels at 353 CpG sites across the human genome, marked a significant breakthrough in the field [1]. Subsequent epigenetic clocks, such as the Hannum clock and the GrimAge clock, have been developed to improve accuracy and predict specific health outcomes, including lifespan and disease risk [2, 3]. GrimAge, for example, incorporates DNA methylation surrogates of plasma proteins and smoking pack-years, providing a more comprehensive assessment of biological age and its relationship with mortality risk. The advantage of epigenetic clocks lies in their relatively high accuracy and their ability to capture long-term cumulative effects of various factors on the aging process. However, epigenetic clocks are relatively expensive due to the need for genome-wide DNA methylation arrays or sequencing. Also, understanding the functional significance of all the CpG sites utilized in the clocks and the mechanisms that lead to age-related DNA methylation changes remains a topic of ongoing research.
2.2 Transcriptomic Signatures
Transcriptomic analysis, which involves measuring the levels of RNA transcripts in a cell or tissue, provides a snapshot of gene expression patterns and reflects the functional state of the organism. Age-related changes in gene expression have been identified in various tissues and cell types, and these changes can be used to develop transcriptomic signatures of biological age. Studies have shown that transcriptomic age can be predicted with reasonable accuracy and that it is associated with age-related diseases [4]. However, transcriptomic signatures can be highly tissue-specific and sensitive to environmental factors, making it challenging to develop a universal transcriptomic clock. Furthermore, the interpretation of transcriptomic data can be complex, requiring sophisticated bioinformatics tools and expertise.
2.3 Proteomic Profiling
Proteomics, the large-scale study of proteins, offers another avenue for assessing biological age. Proteins are the functional molecules of the cell, and their levels and modifications reflect the cellular processes that are most directly affected by aging. Proteomic profiling can identify age-related changes in protein abundance, post-translational modifications, and protein-protein interactions. Studies have identified specific proteins, such as growth differentiation factor 15 (GDF15) and cystatin C, that are strongly associated with aging and mortality risk [5]. Proteomic approaches, particularly those utilizing high-throughput mass spectrometry, are becoming increasingly powerful and affordable, allowing for the simultaneous measurement of thousands of proteins in a single sample. However, proteomic data can be complex and require careful normalization and quality control. The dynamic range of protein concentrations in biological samples can also be challenging, with some proteins being present at very low levels.
2.4 Metabolomic Analysis
Metabolomics, the study of small molecules (metabolites) in a biological sample, provides insights into the metabolic pathways and biochemical processes that are altered during aging. Age-related changes in metabolite levels have been identified in various biofluids, including blood, urine, and cerebrospinal fluid. Metabolomic analysis can identify specific metabolites, such as glucose, lipids, and amino acids, that are associated with aging and disease risk [6]. Metabolomics offers the advantage of being relatively non-invasive and providing a real-time snapshot of metabolic activity. However, metabolite levels can be highly variable and influenced by diet, lifestyle, and medication use, making it challenging to develop robust and reliable metabolomic clocks. Also, the interpretation of metabolomic data can be complex, requiring expertise in biochemistry and pathway analysis.
2.5 Composite Biomarker Indices
Given the limitations of individual biomarkers, researchers have increasingly focused on developing composite biomarker indices that combine multiple measurements to provide a more comprehensive assessment of biological age. These indices typically include a combination of physiological, biochemical, and functional parameters, such as blood pressure, cholesterol levels, glucose tolerance, grip strength, and cognitive function. Examples of composite biomarker indices include the Klemera-Doubal method (KDM) and the PhenoAge clock, which utilizes clinical chemistry parameters to predict lifespan [7]. Composite biomarker indices offer the advantage of integrating information from multiple domains and capturing the complexity of the aging process. However, the selection of appropriate biomarkers and the weighting of their contributions to the index can be challenging. Also, the availability of certain biomarkers may vary across studies and populations.
Many thanks to our sponsor Esdebe who helped us prepare this research report.
3. Determinants of Biological Age
Biological age is not solely determined by genetics but is rather a complex interplay of genetic predispositions, environmental exposures, and lifestyle choices. Understanding the relative contributions of these factors is crucial for developing effective interventions to promote healthy aging.
3.1 Genetic Factors
Genetic factors play a significant role in determining an individual’s biological age trajectory. Studies have identified specific genes and genetic variants that are associated with longevity, age-related diseases, and measures of biological age. For example, variants in the APOE gene, which encodes a protein involved in lipid metabolism, are strongly associated with Alzheimer’s disease risk and have been shown to influence epigenetic age [8]. Genome-wide association studies (GWAS) have identified numerous other genetic variants that are associated with lifespan and healthspan. However, the effect sizes of individual genetic variants are typically small, and the overall contribution of genetics to biological age variation is estimated to be around 20-30% [9]. This suggests that environmental and lifestyle factors play a more dominant role in shaping the aging process. It’s important to note that genes may influence how an individual responds to environmental and lifestyle interventions. A person with certain genetic predispositions may be more susceptible to the negative effects of a poor diet or a sedentary lifestyle, or conversely, may benefit more from healthy lifestyle choices. Future research should focus on understanding gene-environment interactions and developing personalized strategies to promote healthy aging based on an individual’s genetic profile.
3.2 Lifestyle Factors
Lifestyle factors, such as diet, exercise, smoking, and alcohol consumption, have a profound impact on biological age. Numerous studies have demonstrated that healthy lifestyle choices can slow down the aging process and reduce the risk of age-related diseases. Caloric restriction, a dietary intervention that involves reducing calorie intake without causing malnutrition, has been shown to extend lifespan and healthspan in various organisms, including yeast, worms, flies, and mice [10]. In humans, caloric restriction has been shown to improve metabolic health and reduce the risk of cardiovascular disease and type 2 diabetes. Exercise, particularly aerobic exercise and resistance training, has also been shown to have anti-aging effects [11]. Exercise improves cardiovascular function, reduces inflammation, and promotes muscle mass and strength. Smoking and excessive alcohol consumption, on the other hand, are associated with accelerated aging and increased risk of age-related diseases [12]. Smoking damages DNA, impairs immune function, and promotes inflammation. Excessive alcohol consumption can lead to liver damage, cardiovascular disease, and cognitive decline. Adopting a healthy lifestyle, characterized by a balanced diet, regular exercise, and avoidance of smoking and excessive alcohol consumption, is crucial for maintaining a youthful biological age and promoting healthy aging.
3.3 Environmental Factors
Environmental factors, including exposure to pollutants, toxins, and infections, can also influence biological age. Exposure to air pollution, for example, has been shown to accelerate epigenetic aging and increase the risk of cardiovascular disease and respiratory illnesses [13]. Exposure to heavy metals, such as lead and mercury, can damage the nervous system and impair cognitive function. Chronic infections, such as HIV and hepatitis C, can accelerate the aging process and increase the risk of age-related diseases [14]. Adverse childhood experiences, such as abuse and neglect, can also have long-lasting effects on biological age and increase the risk of mental and physical health problems later in life. Creating a healthy environment, characterized by clean air and water, safe food, and access to healthcare, is essential for promoting healthy aging and reducing the burden of age-related diseases.
Many thanks to our sponsor Esdebe who helped us prepare this research report.
4. Biological Age and Disease Risk
One of the most compelling aspects of biological age research is its ability to predict the risk of developing age-related diseases. Studies have consistently shown that individuals with an accelerated biological age are at a higher risk of developing cardiovascular disease, cancer, neurodegenerative disorders, and metabolic syndromes [15]. For example, individuals with a higher epigenetic age are more likely to develop Alzheimer’s disease and other forms of dementia [16]. They are also at increased risk of cardiovascular events, such as heart attack and stroke. Similarly, individuals with a higher PhenoAge, based on clinical chemistry parameters, are at increased risk of mortality from various causes. The relationship between biological age and disease risk is likely mediated by multiple mechanisms, including cellular senescence, inflammation, oxidative stress, and mitochondrial dysfunction. These processes are known to contribute to both aging and disease development. Targeting these processes with interventions aimed at slowing down biological aging may offer a promising strategy for preventing or delaying the onset of age-related diseases.
Many thanks to our sponsor Esdebe who helped us prepare this research report.
5. Interventions to Modulate Biological Age
Given the strong association between biological age and disease risk, there is considerable interest in developing interventions to modulate biological aging and promote healthy aging. Several interventions have shown promise in preclinical studies and are currently being investigated in clinical trials.
5.1 Dietary Restriction and Caloric Restriction Mimetics
As mentioned earlier, dietary restriction and caloric restriction have been shown to extend lifespan and healthspan in various organisms. However, strict caloric restriction can be difficult to maintain in humans and may have adverse side effects. Therefore, researchers are exploring caloric restriction mimetics, which are compounds that mimic the beneficial effects of caloric restriction without requiring a reduction in calorie intake. Resveratrol, a polyphenol found in red wine, and rapamycin, an inhibitor of the mTOR pathway, are examples of caloric restriction mimetics that have shown promise in preclinical studies [17]. Resveratrol has been shown to activate sirtuins, a family of proteins involved in DNA repair and stress resistance. Rapamycin inhibits the mTOR pathway, which regulates cell growth and metabolism. Both resveratrol and rapamycin have been shown to extend lifespan in various organisms and improve metabolic health in humans. However, the long-term effects of these compounds on biological age and disease risk are still under investigation.
5.2 Exercise
Exercise, as previously stated, has numerous health benefits and can slow down the aging process. Exercise improves cardiovascular function, reduces inflammation, and promotes muscle mass and strength. Studies have shown that exercise can improve epigenetic age and reduce the risk of age-related diseases [18]. The type, intensity, and duration of exercise that are most effective for slowing down biological aging are still being investigated. However, a combination of aerobic exercise and resistance training is generally recommended.
5.3 Senolytics and Senomorphics
Cellular senescence, a state of irreversible cell cycle arrest, is a hallmark of aging and contributes to age-related diseases. Senescent cells accumulate in tissues with age and secrete a variety of pro-inflammatory factors that can damage surrounding cells. Senolytics are compounds that selectively eliminate senescent cells, while senomorphics are compounds that suppress the harmful effects of senescent cells. Several senolytics, such as dasatinib and quercetin, have shown promise in preclinical studies and are currently being investigated in clinical trials [19]. These compounds have been shown to improve physical function, reduce inflammation, and extend lifespan in mice. Senomorphics, such as metformin, have also shown promise in preclinical studies and are widely used to treat type 2 diabetes. Metformin has been shown to reduce inflammation and improve metabolic health. The long-term effects of senolytics and senomorphics on biological age and disease risk are still under investigation.
5.4 Emerging Therapies
Several other emerging therapies are being investigated for their potential to modulate biological age. These include stem cell therapy, gene therapy, and epigenetic editing. Stem cell therapy involves transplanting stem cells into damaged tissues to promote regeneration and repair. Gene therapy involves introducing new genes into cells to correct genetic defects or enhance cellular function. Epigenetic editing involves modifying DNA methylation patterns to restore youthful epigenetic profiles. These therapies are still in the early stages of development, but they hold promise for treating age-related diseases and potentially reversing the aging process.
Many thanks to our sponsor Esdebe who helped us prepare this research report.
6. Ethical Considerations
The use of biological age as a predictor of health outcomes raises several ethical considerations. These include the potential for discrimination, the impact on healthcare resource allocation, and the societal perceptions of aging.
6.1 Discrimination
If biological age is used to make decisions about insurance coverage, employment, or access to healthcare, it could lead to discrimination against individuals with an accelerated biological age. Individuals who are deemed to be at higher risk of developing age-related diseases may be denied access to insurance or employment opportunities. This could exacerbate existing health disparities and create new forms of social inequality. It is important to ensure that biological age is used responsibly and ethically and that individuals are not discriminated against based on their biological age.
6.2 Healthcare Resource Allocation
The use of biological age could also influence healthcare resource allocation. If healthcare resources are prioritized for individuals with a younger biological age, it could lead to a neglect of older adults and those with an accelerated biological age. This could widen the gap between the healthy and the unhealthy and create a two-tiered healthcare system. It is important to ensure that healthcare resources are allocated fairly and equitably and that all individuals have access to the care they need, regardless of their biological age.
6.3 Societal Perceptions of Aging
The focus on biological age could also influence societal perceptions of aging. If aging is viewed as a disease that can be treated or reversed, it could lead to a devaluation of older adults and a reinforcement of ageist stereotypes. It is important to promote a positive view of aging and to recognize the value and contributions of older adults to society. The goal should be to promote healthy aging and to improve the quality of life for all individuals, regardless of their chronological or biological age.
Many thanks to our sponsor Esdebe who helped us prepare this research report.
7. Conclusion
Biological age is a powerful predictor of healthspan, lifespan, and the risk of developing age-related diseases. Advances in measurement technologies have enabled the development of increasingly accurate and reliable biomarkers of biological age. Understanding the complex interplay of genetic, lifestyle, and environmental factors that influence biological age is crucial for developing effective interventions to promote healthy aging. Several interventions, including dietary restriction, exercise, senolytics, and emerging therapies, have shown promise in preclinical studies and are being investigated in clinical trials. However, the use of biological age as a predictor of health outcomes raises several ethical considerations that must be carefully addressed to ensure that it is used responsibly and ethically. Future research should focus on further refining the measurement of biological age, identifying new targets for intervention, and evaluating the long-term effects of interventions on healthspan and lifespan. A more nuanced understanding of biological age will enable the development of personalized strategies to promote healthy aging and reduce the burden of age-related diseases.
Many thanks to our sponsor Esdebe who helped us prepare this research report.
References
[1] Horvath, S. (2013). DNA methylation age of human tissues and cell types. Genome Biology, 14(10), R115.
[2] Hannum, G., Guinney, J., Zhao, L., Zhang, L., Hughes, G., Sadda, S., … & Chen, Y. A. (2013). Genome-wide methylation profiles reveal quantitative aging indicators. Molecular Cell, 49(2), 359-367.
[3] Lu, A. T., Quach, A., Hou, L., Chen, B. H., Ghazalpour, A., Ritz, B. R., … & Horvath, S. (2019). DNA methylation GrimAge strongly predicts lifespan and healthspan. Aging, 11(2), 1583-1600.
[4] Peters, M. J., Joehanes, R., Pilling, L. C., Schurmann, C., Conneely, K. N., Powell, J., … & Levy, D. (2015). The transcriptional landscape of age in human peripheral blood. Nature Communications, 6(1), 8570.
[5] Tanisawa, K., Arai, Y., Izumi, S., Abe, Y., Takayama, M., Murayama, H., … & Hirose, N. (2015). Serum GDF15 is associated with aging and mortality in community-dwelling older adults. Scientific Reports, 5(1), 1-9.
[6] Gonzalez-Freire, M., & Barcena, J. A. (2019). Metabolomics in aging and longevity. Current Opinion in Biotechnology, 56, 108-114.
[7] Levine, M. E., Lu, A. T., Quach, A., Chen, B. H., Assimes, T. L., Bandinelli, S., … & Horvath, S. (2018). An epigenetic biomarker of aging for lifespan and healthspan. Aging, 10(4), 573.
[8] Levine, M. E., Lu, A. T., Chen, B. H., Hernandez, D. G., Singleton, A. B., Ferrucci, L., … & Horvath, S. (2015). Epigenetic age of the brain is associated with Alzheimer’s disease pathology. Aging, 7(12), 1183.
[9] Christensen, K., McGue, M., Petersen, I., Jeune, B., & Vaupel, J. W. (2006). Genetics of human longevity: what have we learned so far?. Trends in Genetics, 22(2), 87-92.
[10] Fontana, L., Partridge, L., & Longo, V. D. (2010). Extending healthy life span–from yeast to humans. Science, 328(5976), 321-326.
[11] Garatachea, N., Lucia, A., Emanuele, E., & Santiago, C. (2015). Exercise, aging and the telomere connection. Exp Gerontol, 68, 1-10.
[12] Reuter, S., Gupta, S. C., Chaturvedi, M. M., & Aggarwal, B. B. (2010). Oxidative stress, inflammation, and cancer: how are they linked?. Free Radical Biology and Medicine, 49(11), 1603-1616.
[13] Breitner, S., Hampel, R., Wolf, K., Rückerl, R., Peters, A., & Schneider, A. (2016). Air pollution and epigenetic age acceleration. Environmental Health Perspectives, 124(11), 1620-1625.
[14] Valenzuela, H. F., & Effros, R. B. (2013). Telomeres and T cells: cause, consequence, or cure for aging?. Journal of Immunology, 190(10), 4859-4864.
[15] Jylhävä, J., Pedersen, N. L., & Hägg, S. (2017). Biological age and its estimation using biomarkers. Journal of Internal Medicine, 283(5), 482-503.
[16] Levine, M. E., Lu, A. T., Chen, B. H., Hernandez, D. G., Singleton, A. B., Ferrucci, L., … & Horvath, S. (2015). Epigenetic age of the brain is associated with Alzheimer’s disease pathology. Aging, 7(12), 1183-1190.
[17] de Cabo, R., Carmona-Gutierrez, D., Bernier, M., Hall, M. N., Madeo, F., & Kroemer, G. (2014). The search for antiaging interventions: from elixirs to mimetics. Cell, 157(7), 1515-1526.
[18] Zaninotto, P., Batty, G. D., Allerhand, M., & Deary, I. J. (2018). Association of grip strength with type 2 diabetes: prospective evidence from the English Longitudinal Study of Ageing. Age and Ageing, 47(1), 80-86.
[19] Kirkland, J. L., Tchkonia, T., Zhu, Y., van Deursen, J. M., & Curiel, T. J. (2017). The clinical potential of senolytic drugs. Journal of the American Geriatrics Society, 65(10), 2297-2307.
The discussion on ethical considerations is crucial, particularly regarding potential discrimination based on biological age in areas like insurance and employment. How can we proactively establish safeguards to prevent such biases and ensure equitable access, regardless of one’s biological aging trajectory?
That’s a great point! Establishing proactive safeguards against discrimination is essential. Perhaps a standardized ethical framework, developed through public discourse and expert consensus, could help guide the responsible use of biological age data and ensure equitable access in various sectors. What are your thoughts on the role of policy makers in this process?
Editor: MedTechNews.Uk
Thank you to our Sponsor Esdebe
The report mentions composite biomarker indices. How might the integration of AI and machine learning enhance the precision and predictive power of these indices by identifying novel biomarker combinations or refining existing algorithms?
That’s an insightful question! AI and machine learning could revolutionize composite biomarker indices. By analyzing vast datasets, AI might uncover subtle, previously unknown correlations between biomarkers, leading to more accurate biological age predictions and a deeper understanding of aging. Imagine personalized indices that adapt based on individual health data!
Editor: MedTechNews.Uk
Thank you to our Sponsor Esdebe
This is a fascinating report. The discussion on lifestyle factors influencing biological age, particularly diet and exercise, highlights actionable areas for individuals to potentially improve their healthspans. Are there studies exploring the synergistic effects of combining multiple lifestyle interventions?