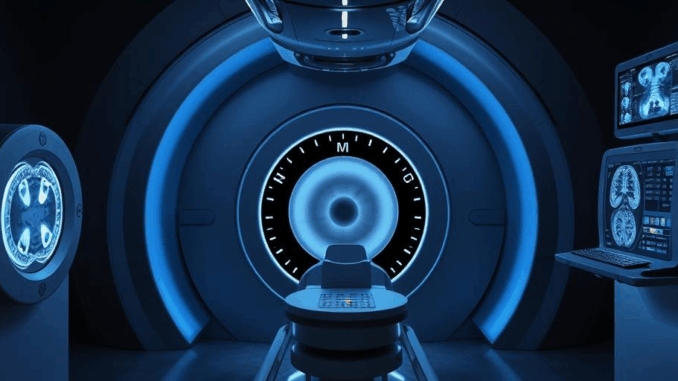
Abstract
Computed Tomography (CT) has revolutionized medical imaging, providing detailed cross-sectional views of the human body. This report offers a comprehensive overview of CT technology, tracing its evolution from early axial scanners to modern multi-detector and spectral CT systems. It delves into the fundamental principles of CT image formation, including X-ray physics, data acquisition methods, and image reconstruction algorithms. A detailed discussion of different CT modalities, such as single-energy, dual-energy, and photon-counting CT, is presented, highlighting their respective advantages and limitations. The report also addresses the critical aspects of radiation dose management in CT, exploring techniques like automatic exposure control and iterative reconstruction. Furthermore, we examine the application of CT in various clinical domains, extending beyond lung cancer detection to encompass cardiovascular imaging, neurological assessments, musculoskeletal evaluations, and abdominal pathologies. Finally, the report explores emerging trends in CT research, including artificial intelligence integration for image analysis and dose optimization, paving the way for future advancements in this vital imaging modality.
Many thanks to our sponsor Esdebe who helped us prepare this research report.
1. Introduction
Computed Tomography (CT) has become an indispensable tool in modern medicine, enabling clinicians to visualize internal anatomy with unprecedented detail. Its ability to acquire high-resolution, cross-sectional images has significantly improved diagnostic accuracy and patient management across a wide range of medical specialties. Unlike conventional radiography, which provides two-dimensional projections of overlapping structures, CT employs sophisticated algorithms to reconstruct three-dimensional images from multiple X-ray projections, allowing for precise localization and characterization of pathological processes.
The evolution of CT technology has been rapid and transformative. The first clinical CT scanner, developed by Sir Godfrey Hounsfield in the early 1970s, utilized a single X-ray tube and detector to acquire data in an axial, step-and-shoot manner. This early system required lengthy scan times and produced relatively low-resolution images. Over the subsequent decades, significant advancements have led to the development of multi-detector CT (MDCT) scanners, which utilize multiple detector rows to acquire data simultaneously, dramatically reducing scan times and improving image quality. More recent innovations, such as dual-energy CT (DECT) and photon-counting CT (PCCT), have further expanded the capabilities of CT imaging, providing additional diagnostic information and reducing radiation dose.
This report aims to provide a comprehensive overview of CT technology, covering its fundamental principles, different modalities, radiation dose considerations, and clinical applications. We will delve into the technical aspects of CT image formation, discussing the physics of X-ray interactions, data acquisition methods, and image reconstruction algorithms. We will also examine the advantages and limitations of different CT modalities, such as single-energy, dual-energy, and photon-counting CT. Furthermore, we will address the critical issue of radiation dose management in CT, exploring various techniques for reducing patient exposure. Finally, we will discuss the role of CT in diagnosing and managing a wide range of diseases, highlighting its impact on clinical practice.
Many thanks to our sponsor Esdebe who helped us prepare this research report.
2. Principles of CT Image Formation
The formation of CT images relies on the principles of X-ray attenuation and mathematical reconstruction. When an X-ray beam passes through the body, photons interact with the tissues, resulting in a reduction in the intensity of the beam. The extent of attenuation depends on the energy of the X-ray photons and the density and atomic composition of the tissue. Tissues with high density and high atomic number, such as bone, attenuate more X-rays than tissues with low density and low atomic number, such as air.
The attenuated X-ray beam is measured by detectors, which convert the X-ray energy into electrical signals. These signals are then digitized and processed by a computer to create a set of projection data. Each projection represents the attenuation profile of the X-ray beam along a specific path through the body. To create a CT image, multiple projections are acquired from different angles around the patient.
2.1. X-ray Physics and Attenuation
The interaction of X-rays with matter is governed by several physical processes, including photoelectric absorption, Compton scattering, and Rayleigh scattering. Photoelectric absorption occurs when an X-ray photon is completely absorbed by an atom, resulting in the ejection of an electron. This process is dominant at low X-ray energies and is highly dependent on the atomic number of the tissue. Compton scattering occurs when an X-ray photon interacts with an electron, resulting in the ejection of the electron and a change in the direction of the photon. This process is dominant at intermediate X-ray energies and is less dependent on the atomic number of the tissue. Rayleigh scattering occurs when an X-ray photon interacts with an atom without losing energy, resulting in a change in the direction of the photon. This process is relatively minor in CT imaging.
The attenuation of an X-ray beam as it passes through the body can be described by the Beer-Lambert law:
I = I₀ * exp(-μx)
where I is the intensity of the transmitted X-ray beam, I₀ is the intensity of the incident X-ray beam, μ is the linear attenuation coefficient of the tissue, and x is the thickness of the tissue.
The linear attenuation coefficient (μ) is a measure of how much X-rays are attenuated per unit length of tissue. It depends on the energy of the X-ray photons and the density and atomic composition of the tissue. In CT imaging, the linear attenuation coefficient is converted to a CT number, which is a standardized measure of tissue density. CT numbers are typically expressed in Hounsfield units (HU), with water assigned a value of 0 HU and air assigned a value of -1000 HU.
2.2. Data Acquisition Methods
CT data acquisition involves rotating an X-ray tube and detector array around the patient to acquire multiple projections. Different generations of CT scanners have employed different data acquisition methods. First-generation CT scanners used a single X-ray tube and detector, which translated across the patient and then rotated by a small angle. This process was repeated until a complete set of projections was acquired. Second-generation CT scanners used a fan-shaped X-ray beam and a linear array of detectors, which translated across the patient and then rotated by a small angle. Third-generation CT scanners used a fan-shaped X-ray beam and a curved array of detectors, which rotated around the patient. Fourth-generation CT scanners used a fan-shaped X-ray beam and a stationary ring of detectors, with only the X-ray tube rotating around the patient.
Modern MDCT scanners utilize multiple detector rows to acquire data simultaneously. This allows for faster scan times and improved image quality. MDCT scanners typically use a cone-shaped X-ray beam and a two-dimensional array of detectors. The detector array is divided into multiple rows, each of which acquires a separate set of projections. The number of detector rows determines the number of slices that can be acquired per rotation. For example, a 64-slice CT scanner has 64 detector rows, which allows it to acquire 64 slices per rotation.
2.3. Image Reconstruction Algorithms
CT images are reconstructed from the projection data using mathematical algorithms. The most commonly used reconstruction algorithm is filtered back-projection (FBP). FBP involves filtering the projection data to remove blurring artifacts and then back-projecting the filtered data onto an image grid. The back-projection process involves summing the contributions from all projections at each point in the image grid. The resulting image represents the distribution of CT numbers within the scanned volume.
FBP is a computationally efficient algorithm, but it can be susceptible to artifacts, particularly when the projection data is incomplete or noisy. Iterative reconstruction algorithms have been developed to address these limitations. Iterative reconstruction algorithms involve iteratively refining an initial image estimate until it converges to a solution that is consistent with the projection data. These algorithms are more computationally intensive than FBP, but they can produce higher-quality images with reduced artifacts. Several different iterative reconstruction algorithms are available, including algebraic reconstruction technique (ART), simultaneous iterative reconstruction technique (SIRT), and model-based iterative reconstruction (MBIR). MBIR algorithms incorporate detailed models of the CT scanner and the patient’s anatomy to improve image quality and reduce radiation dose.
Many thanks to our sponsor Esdebe who helped us prepare this research report.
3. CT Modalities: Single-Energy, Dual-Energy, and Photon-Counting CT
3.1 Single-Energy CT (SECT)
Single-energy CT (SECT) is the most conventional form of CT imaging. In SECT, the X-ray tube emits photons within a broad energy spectrum characterized by a single peak energy value (e.g., 120 kVp). The detectors then measure the total number of photons transmitted through the patient, without distinguishing between the energies of individual photons. The resulting images reflect the average attenuation properties of tissues at the selected X-ray energy.
SECT is widely used for a broad range of clinical applications, including the diagnosis of fractures, infections, tumors, and vascular abnormalities. The primary advantages of SECT include its wide availability, relatively low cost, and ease of implementation. However, SECT has limitations in differentiating tissues with similar densities but different compositions. For example, it can be difficult to distinguish between iodine contrast and calcifications on SECT images, as both substances exhibit high attenuation at typical X-ray energies.
3.2. Dual-Energy CT (DECT)
Dual-energy CT (DECT) overcomes some of the limitations of SECT by acquiring data at two different X-ray energy levels. This can be achieved using several different techniques, including dual-source CT, rapid kVp switching, and split-filter CT. Dual-source CT systems use two X-ray tubes operating at different energy levels, allowing for simultaneous acquisition of data at two different energies. Rapid kVp switching systems rapidly switch the X-ray tube voltage between two different levels, acquiring data at two different energies in rapid succession. Split-filter CT systems use a filter to split the X-ray beam into two beams with different energy spectra.
By acquiring data at two different energy levels, DECT can provide additional information about the composition of tissues. This allows for improved differentiation of tissues with similar densities but different compositions. For example, DECT can be used to distinguish between iodine contrast and calcifications, as iodine exhibits a greater change in attenuation with energy than calcifications. DECT can also be used to quantify the amount of iodine contrast in tissues, which can be useful for evaluating tumor vascularity and perfusion.
DECT has a wide range of clinical applications, including the detection of gout, the characterization of renal stones, the evaluation of pulmonary embolism, and the assessment of bone marrow edema. The primary advantages of DECT include its improved tissue differentiation capabilities and its ability to quantify tissue composition. However, DECT is more complex and expensive than SECT, and it typically requires higher radiation doses.
3.3. Photon-Counting CT (PCCT)
Photon-counting CT (PCCT) represents a significant advancement in CT technology. Unlike conventional CT detectors, which measure the total energy deposited by X-ray photons, PCCT detectors count individual photons and measure their energy. This allows for improved energy resolution and reduced electronic noise.
PCCT detectors are typically made of semiconductor materials, such as cadmium telluride (CdTe) or cadmium zinc telluride (CdZnTe). These materials convert X-ray photons directly into electrical signals, without the need for a scintillator. PCCT detectors can be designed with multiple energy thresholds, allowing for the separation of photons into different energy bins. This allows for the acquisition of spectral information, which can be used to improve tissue differentiation and reduce artifacts.
PCCT has the potential to significantly improve CT image quality and reduce radiation dose. The improved energy resolution of PCCT can lead to better tissue differentiation and reduced artifacts. The reduced electronic noise of PCCT can allow for lower radiation doses. PCCT also enables new imaging techniques, such as quantitative spectral imaging, which can provide detailed information about the composition of tissues.
PCCT is still a relatively new technology, but it is rapidly being developed and commercialized. Several PCCT scanners are now available for clinical use. PCCT has shown promising results in a variety of clinical applications, including cardiovascular imaging, oncology, and musculoskeletal imaging. The main challenges facing PCCT include its higher cost and its limited detector size. However, as the technology matures, PCCT is expected to become an increasingly important tool in medical imaging.
Many thanks to our sponsor Esdebe who helped us prepare this research report.
4. Radiation Dose Management in CT
Radiation exposure is a significant concern in CT imaging. CT scans deliver a higher radiation dose than conventional X-ray examinations, and repeated CT scans can increase the risk of developing cancer. Therefore, it is essential to minimize radiation dose in CT imaging while maintaining diagnostic image quality. This is underpinned by the ALARA (As Low As Reasonably Achievable) principle.
4.1. Factors Affecting Radiation Dose
Several factors affect the radiation dose in CT imaging, including the tube voltage (kVp), tube current (mA), scan time, pitch, and collimation. Higher tube voltages and tube currents result in higher radiation doses. Longer scan times also increase radiation dose. Pitch is the distance the patient table moves per rotation of the X-ray tube. Higher pitch values result in faster scan times but also lower image quality and higher radiation doses. Collimation is the width of the X-ray beam. Narrower collimation reduces scatter radiation but also requires higher tube currents to maintain image quality.
4.2. Techniques for Reducing Radiation Dose
Several techniques can be used to reduce radiation dose in CT imaging, including automatic exposure control (AEC), iterative reconstruction, and organ-based tube current modulation. AEC automatically adjusts the tube current based on the patient’s size and attenuation characteristics. This helps to ensure that the image quality is consistent across patients of different sizes. Iterative reconstruction algorithms can produce higher-quality images with lower radiation doses than FBP. Organ-based tube current modulation reduces the tube current when scanning radiosensitive organs, such as the thyroid and gonads.
Specific strategies include:
- Justification of the examination: Ensuring the clinical benefit outweighs the radiation risk.
- Optimization of imaging parameters: Tailoring kVp, mA, pitch, and collimation to the specific clinical indication and patient size.
- Use of shielding: Protecting radiosensitive organs, such as the thyroid and gonads, with lead shields.
- Education and training: Ensuring that CT technologists are properly trained in radiation dose reduction techniques.
4.3. Low-Dose CT Protocols
Low-dose CT protocols have been developed for specific applications, such as lung cancer screening and renal stone detection. These protocols use lower tube currents and voltages to reduce radiation dose. Low-dose CT protocols can significantly reduce radiation dose without compromising diagnostic image quality. For example, low-dose CT lung cancer screening has been shown to reduce lung cancer mortality by up to 20% in high-risk individuals, while delivering a relatively low radiation dose.
Many thanks to our sponsor Esdebe who helped us prepare this research report.
5. Clinical Applications of CT
CT has a wide range of clinical applications, spanning nearly every medical specialty. Its ability to provide detailed cross-sectional images of the human body has revolutionized the diagnosis and management of a vast array of diseases. The uses are only limited by the availability of scanners and qualified operators. Specific applications are only limited by the skill of the requesting clinician.
5.1. Cardiovascular Imaging
CT is increasingly used for cardiovascular imaging, including the detection of coronary artery disease, the evaluation of aortic aneurysms, and the assessment of pulmonary embolism. CT angiography (CTA) is a non-invasive technique that uses intravenous contrast to visualize the arteries. CTA can be used to detect and quantify coronary artery stenosis, which is a narrowing of the coronary arteries. CTA can also be used to evaluate aortic aneurysms, which are bulges in the wall of the aorta. CT pulmonary angiography (CTPA) is used to diagnose pulmonary embolism, which is a blood clot in the lungs.
5.2. Neurological Assessments
CT is a valuable tool for neurological assessments, including the evaluation of stroke, trauma, and brain tumors. CT can be used to detect acute ischemic stroke, which is a blockage of blood flow to the brain. CT can also be used to evaluate head trauma, including skull fractures and intracranial hemorrhage. CT can be used to detect and characterize brain tumors.
5.3. Musculoskeletal Evaluations
CT is used for musculoskeletal evaluations, including the detection of fractures, infections, and tumors. CT can be used to detect subtle fractures that may not be visible on conventional X-rays. CT can also be used to evaluate bone infections, such as osteomyelitis. CT can be used to detect and characterize bone tumors.
5.4. Abdominal Pathologies
CT is widely used for the evaluation of abdominal pathologies, including the detection of liver tumors, kidney stones, and appendicitis. CT can be used to detect and characterize liver tumors, such as hepatocellular carcinoma. CT can be used to detect kidney stones and to evaluate their size and location. CT can be used to diagnose appendicitis, which is an inflammation of the appendix.
5.5 Oncological Imaging
The role of CT in oncological imaging is paramount in staging cancer, monitoring treatment response and detecting recurrence. It is also used for treatment planning.
Many thanks to our sponsor Esdebe who helped us prepare this research report.
6. Emerging Trends in CT Research
CT technology is constantly evolving, with ongoing research focused on improving image quality, reducing radiation dose, and expanding clinical applications. Several emerging trends in CT research are particularly noteworthy.
6.1. Artificial Intelligence (AI) Integration
AI is increasingly being integrated into CT imaging, with applications in image reconstruction, image analysis, and dose optimization. AI algorithms can be used to improve the quality of CT images, reduce artifacts, and enhance the detection of subtle lesions. AI algorithms can also be used to automate image analysis tasks, such as lesion segmentation and quantification. Furthermore, AI can be used to optimize radiation dose by predicting the optimal scanning parameters for each patient.
6.2. Advanced Reconstruction Techniques
Advanced reconstruction techniques, such as deep learning-based reconstruction, are being developed to further improve image quality and reduce radiation dose. These techniques leverage the power of deep neural networks to learn complex relationships between the projection data and the reconstructed image. Deep learning-based reconstruction algorithms can produce images with higher resolution and lower noise levels than traditional reconstruction algorithms.
6.3. Spectral Photon-Counting CT
Spectral photon-counting CT (SPCCT) is an emerging technology that has the potential to revolutionize CT imaging. SPCCT detectors count individual photons and measure their energy, allowing for the acquisition of detailed spectral information. SPCCT can provide improved tissue differentiation, reduced artifacts, and new imaging capabilities, such as quantitative spectral imaging. Ongoing research is focused on developing SPCCT detectors with higher energy resolution and larger detector sizes.
Many thanks to our sponsor Esdebe who helped us prepare this research report.
7. Conclusion
Computed Tomography has transformed medical imaging, providing detailed cross-sectional views of the human body and enabling clinicians to diagnose and manage a wide range of diseases. From its early axial scanners to modern multi-detector and spectral CT systems, CT technology has undergone rapid and transformative advancements. The fundamental principles of CT image formation, including X-ray physics, data acquisition methods, and image reconstruction algorithms, are critical to understanding the capabilities and limitations of this vital imaging modality. The development of different CT modalities, such as single-energy, dual-energy, and photon-counting CT, has further expanded the diagnostic information available to clinicians. Radiation dose management remains a critical aspect of CT imaging, and ongoing research is focused on developing techniques to minimize patient exposure while maintaining diagnostic image quality. Finally, the integration of artificial intelligence into CT imaging holds great promise for improving image quality, automating image analysis tasks, and optimizing radiation dose. As CT technology continues to evolve, it will undoubtedly play an increasingly important role in medical imaging and patient care.
Many thanks to our sponsor Esdebe who helped us prepare this research report.
References
- Bushberg, J. T., Seibert, J. A., Leidholdt, E. M., Jr, & Boone, J. M. (2021). The Essential Physics of Medical Imaging (4th ed.). Wolters Kluwer.
- D.L. McDaniel, W.W. Orrison, CT physics: the basics, Eur. J. Radiol. 85 (8) (2016) 1707–1712.
- Flohr, T. G., McCollough, C. H., Bruder, H., Petersilka, M., Gruber, K., Süss, C., … & Stierstorfer, K. (2006). First performance evaluation of a dual-source CT (DSCT) system. European Radiology, 16(2), 256-268.
- Greffier, J., Hamard, A., Vakalopoulou, M., Facon, D., & Allado, E. (2020). Artificial intelligence in CT imaging. Diagnostic and Interventional Imaging, 101(11), 647-655.
- McCollough, C. H., Bushberg, J. T., Fletcher, J. G., & Payne, J. T. (2015). Answers to common questions about CT radiation dose. American Journal of Roentgenology, 204(6), 1163-1172.
- Taguchi, K., & Iwanczyk, J. S. (2013). Vision 20/20: single photon counting detectors in medical imaging. Medical Physics, 40(10), 100901.
- Yu, L., Christner, J. A., Leng, S., Wang, J., Fletcher, J. G., & McCollough, C. H. (2009). Dual-energy and spectral CT: general principles. American Journal of Roentgenology, 192(6), 1399-1415.
The integration of AI for image analysis is a promising avenue. How might these AI algorithms be trained to differentiate between clinically significant anomalies and benign variations, thereby reducing false positives and improving diagnostic accuracy?
That’s a great question! One approach is to train AI models on large, carefully curated datasets that include both clinically significant anomalies and a wide spectrum of benign variations, with expert radiologists providing detailed annotations. Another is to use a multi-stage AI pipeline where one model identifies potential anomalies and another filters out benign ones. Open to other suggestions!
Editor: MedTechNews.Uk
Thank you to our Sponsor Esdebe
AI optimizing radiation dose? So, the machines are now deciding how much zap we get? I hope they’ve seen enough sci-fi movies to know what *not* to do. Perhaps they should start with a course in bedside manner too.