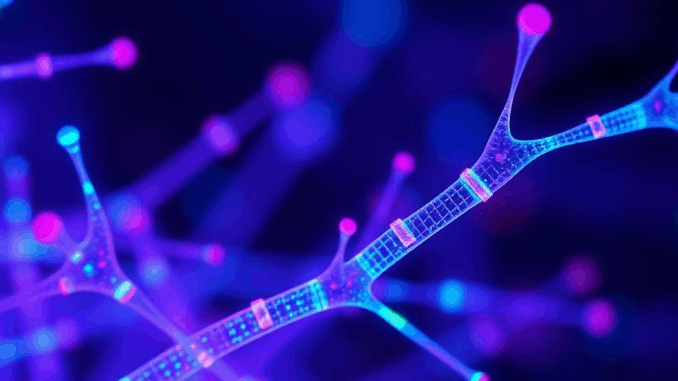
Abstract
Fluorophores are molecules that exhibit fluorescence, a phenomenon of emitting light upon excitation by electromagnetic radiation. This property has made them indispensable tools across diverse scientific disciplines, from bioimaging and diagnostics to materials science and environmental monitoring. This review provides a comprehensive overview of fluorophores, encompassing their chemical structures, photophysical properties, and various classes, including organic dyes, fluorescent proteins, quantum dots, and lanthanide complexes. We delve into the underlying mechanisms of fluorescence, exploring the factors that influence fluorescence intensity, such as pH, temperature, solvent polarity, and quenching phenomena. Furthermore, we discuss the applications of fluorophores in bioimaging, diagnostics, theranostics, and other fields. The review also addresses the limitations associated with fluorophores, particularly photobleaching and toxicity, and highlights recent advancements in the development of novel fluorophores with improved properties, such as enhanced brightness, photostability, and biocompatibility. The integration of fluorophores with nanomaterials, including nanoclays, for targeted delivery and enhanced imaging sensitivity is also discussed. Finally, we offer perspectives on future directions in fluorophore research, focusing on the development of stimuli-responsive fluorophores, near-infrared emitting fluorophores, and biocompatible fluorophores for advanced biological and biomedical applications.
Many thanks to our sponsor Esdebe who helped us prepare this research report.
1. Introduction
Fluorescence, the emission of light by a substance that has absorbed light or other electromagnetic radiation, is a powerful phenomenon utilized extensively across a wide range of scientific disciplines. The molecules that exhibit this property are called fluorophores or fluorescent dyes. Their ability to absorb light at one wavelength and emit light at a longer wavelength has made them invaluable tools in bioimaging, diagnostics, materials science, and environmental monitoring. The fundamental principle underlying fluorescence involves the excitation of a fluorophore’s electron to a higher energy level upon absorption of light, followed by the rapid relaxation of the electron back to the ground state, releasing energy in the form of a photon of light. The difference in energy between the absorbed photon and the emitted photon is known as the Stokes shift, which is a characteristic feature of each fluorophore.
The evolution of fluorophore research has been marked by continuous efforts to develop molecules with improved properties, such as enhanced brightness, photostability, biocompatibility, and spectral tunability. Early fluorophores, primarily organic dyes, suffered from limitations such as poor photostability and broad emission spectra. However, advancements in synthetic chemistry and materials science have led to the development of novel fluorophores, including fluorescent proteins, quantum dots (QDs), and lanthanide complexes, which exhibit superior performance characteristics. Fluorescent proteins, discovered in marine organisms, revolutionized bioimaging by enabling the visualization of cellular processes in real-time and in vivo. Quantum dots, semiconductor nanocrystals, offer exceptional brightness, narrow emission spectra, and high photostability, making them ideal for multiplexed imaging and long-term tracking studies. Lanthanide complexes, characterized by their large Stokes shifts and long emission lifetimes, are particularly useful for time-resolved fluorescence imaging, which minimizes background interference.
Recently, the integration of fluorophores with nanomaterials has emerged as a promising strategy for enhancing their performance and expanding their applications. Nanomaterials, such as nanoclays, nanoparticles, and nanotubes, can serve as carriers for fluorophores, providing protection from degradation, improving their biocompatibility, and enabling targeted delivery to specific sites. Furthermore, the interaction between fluorophores and nanomaterials can lead to novel photophysical properties, such as enhanced fluorescence intensity and altered emission spectra. This review aims to provide a comprehensive overview of fluorophores, encompassing their chemical structures, photophysical properties, various classes, applications, limitations, and future directions. We will explore the underlying mechanisms of fluorescence, discuss the factors that influence fluorescence intensity, and highlight recent advancements in the development of novel fluorophores with improved properties.
Many thanks to our sponsor Esdebe who helped us prepare this research report.
2. Chemical Structure and Photophysical Properties
The chemical structure of a fluorophore is intimately linked to its photophysical properties, which determine its excitation and emission spectra, fluorescence quantum yield, and photostability. Most fluorophores contain aromatic or heterocyclic ring systems with conjugated π-electrons, which are responsible for the absorption and emission of light. The presence of electron-donating or electron-withdrawing groups can influence the energy levels of the π-electrons, thereby modulating the excitation and emission wavelengths.
The process of fluorescence involves several key steps. First, the fluorophore absorbs a photon of light, which promotes an electron from the ground state (S0) to an excited state (S1, S2, etc.). This process is extremely rapid, typically occurring within femtoseconds (10-15 s). The excited electron then undergoes vibrational relaxation, losing energy to the surrounding environment and reaching the lowest vibrational level of the S1 state. From this state, the electron can return to the ground state via several pathways. Fluorescence emission occurs when the electron returns to the ground state by emitting a photon of light. This process is typically on the nanosecond timescale (10-9 s). Other pathways for returning to the ground state include non-radiative decay, such as internal conversion and intersystem crossing. Internal conversion involves the transition from one excited state to another of the same spin multiplicity (e.g., S1 to S0), while intersystem crossing involves the transition from a singlet excited state (S1) to a triplet excited state (T1). Once in the triplet state, the electron can return to the ground state via phosphorescence, which is a much slower process than fluorescence, or via non-radiative decay.
The fluorescence quantum yield (Φ) is a measure of the efficiency of fluorescence emission, defined as the ratio of the number of photons emitted to the number of photons absorbed. A fluorophore with a high quantum yield emits a large number of photons for each photon absorbed, making it a bright and easily detectable probe. The fluorescence lifetime (τ) is the average time that a fluorophore spends in the excited state before returning to the ground state. It is inversely proportional to the rate of fluorescence decay and is influenced by both radiative and non-radiative decay processes. The fluorescence lifetime is an intrinsic property of a fluorophore and can be used to distinguish between different fluorophores in a mixture or to probe the local environment surrounding the fluorophore.
Many thanks to our sponsor Esdebe who helped us prepare this research report.
3. Different Classes of Fluorophores
Fluorophores can be broadly classified into several categories, including organic dyes, fluorescent proteins, quantum dots, and lanthanide complexes. Each class possesses unique properties that make them suitable for specific applications.
3.1 Organic Dyes
Organic dyes are the most traditional type of fluorophores and encompass a wide range of chemical structures, including coumarins, rhodamines, cyanines, and BODIPYs (boron-dipyrromethenes). They are relatively easy to synthesize and modify, allowing for the fine-tuning of their photophysical properties. Organic dyes typically exhibit high extinction coefficients, meaning they absorb light strongly, but their fluorescence quantum yields and photostabilities can vary significantly depending on their structure and environment. Rhodamine derivatives, such as rhodamine 6G and tetramethylrhodamine (TAMRA), are widely used in bioimaging due to their high brightness and good photostability. Cyanine dyes, such as Cy3 and Cy5, are popular choices for fluorescence microscopy and flow cytometry because their emission wavelengths span the visible and near-infrared regions. BODIPY dyes are known for their narrow emission spectra, high fluorescence quantum yields, and chemical stability, making them attractive for various applications, including biosensing and labeling.
3.2 Fluorescent Proteins
Fluorescent proteins (FPs), such as green fluorescent protein (GFP) and its derivatives, are genetically encodable fluorophores that have revolutionized bioimaging. They are derived from marine organisms and consist of a protein structure that undergoes autocatalytic cyclization to form a chromophore, which is responsible for the fluorescence. FPs can be fused to target proteins, allowing for the visualization of protein localization, dynamics, and interactions in living cells. GFP exhibits green fluorescence, while its derivatives, such as enhanced GFP (EGFP), cyan fluorescent protein (CFP), and yellow fluorescent protein (YFP), emit light at different wavelengths. The development of red fluorescent proteins (RFPs), such as mCherry and mKate, has expanded the possibilities for multicolor imaging. FPs are generally biocompatible and can be expressed in a wide range of organisms, making them powerful tools for biological research.
3.3 Quantum Dots
Quantum dots (QDs) are semiconductor nanocrystals that exhibit size-dependent optical properties. Their emission wavelength can be tuned by controlling their size and composition. QDs possess several advantages over traditional fluorophores, including high brightness, narrow emission spectra, broad excitation spectra, and high photostability. They are typically composed of a core material, such as cadmium selenide (CdSe) or indium phosphide (InP), and a shell material, such as zinc sulfide (ZnS), which enhances their stability and fluorescence quantum yield. QDs can be conjugated to antibodies, peptides, or other targeting molecules for specific labeling of cells and tissues. Their high brightness and photostability make them ideal for long-term tracking studies and multiplexed imaging. However, the potential toxicity of QDs, particularly those containing cadmium, remains a concern, and efforts are underway to develop less toxic QDs based on alternative materials.
3.4 Lanthanide Complexes
Lanthanide complexes, also known as lanthanide chelates, consist of a lanthanide ion, such as europium (Eu3+) or terbium (Tb3+), coordinated to organic ligands. They exhibit unique photophysical properties, including large Stokes shifts, long emission lifetimes, and narrow emission bands. The large Stokes shifts minimize background interference, while the long emission lifetimes allow for time-resolved fluorescence imaging, which further reduces background noise. Lanthanide complexes are particularly useful for bioassays and diagnostics, where high sensitivity and low background are essential. However, their relatively low extinction coefficients and quantum yields can limit their brightness, and their emission spectra are often sensitive to the surrounding environment.
Many thanks to our sponsor Esdebe who helped us prepare this research report.
4. Mechanisms of Fluorescence
The mechanism of fluorescence involves several key steps, including light absorption, vibrational relaxation, internal conversion, intersystem crossing, and fluorescence emission. Understanding these processes is crucial for designing and optimizing fluorophores for specific applications.
When a fluorophore absorbs a photon of light, an electron is promoted from the ground state (S0) to an excited singlet state (S1, S2, etc.). The energy of the absorbed photon must match the energy difference between the ground state and the excited state. The excited electron then undergoes vibrational relaxation, losing energy to the surrounding environment and reaching the lowest vibrational level of the S1 state. This process is very fast, typically occurring within picoseconds (10-12 s). From this state, the electron can return to the ground state via several pathways.
Fluorescence emission occurs when the electron returns to the ground state by emitting a photon of light. The energy of the emitted photon is lower than the energy of the absorbed photon, resulting in a Stokes shift. The Stokes shift is due to the energy lost during vibrational relaxation and other non-radiative processes. The fluorescence lifetime is the average time that a fluorophore spends in the excited state before returning to the ground state. It is influenced by both radiative and non-radiative decay processes.
Other pathways for returning to the ground state include internal conversion and intersystem crossing. Internal conversion involves the transition from one excited state to another of the same spin multiplicity (e.g., S1 to S0). This process does not involve the emission of light and can reduce the fluorescence quantum yield. Intersystem crossing involves the transition from a singlet excited state (S1) to a triplet excited state (T1). Once in the triplet state, the electron can return to the ground state via phosphorescence, which is a much slower process than fluorescence, or via non-radiative decay. The triplet state is also more susceptible to reactions with other molecules, such as oxygen, leading to the formation of free radicals and photobleaching.
Many thanks to our sponsor Esdebe who helped us prepare this research report.
5. Factors Affecting Fluorescence Intensity
Fluorescence intensity is influenced by several factors, including pH, temperature, solvent polarity, and quenching phenomena. Understanding these factors is essential for accurate interpretation of fluorescence data and for optimizing experimental conditions.
5.1 pH
The pH of the environment can significantly affect the fluorescence intensity of certain fluorophores, particularly those containing ionizable groups, such as carboxylic acids or amines. Changes in pH can alter the protonation state of these groups, which can affect the electron distribution and the photophysical properties of the fluorophore. For example, some fluorophores exhibit increased fluorescence intensity at acidic pH due to protonation of an amine group, while others exhibit decreased fluorescence intensity at acidic pH due to protonation of a carboxylic acid group. pH-sensitive fluorophores can be used as indicators to measure pH changes in cells and tissues.
5.2 Temperature
Temperature can also influence fluorescence intensity. In general, fluorescence intensity decreases with increasing temperature. This is because higher temperatures promote non-radiative decay processes, such as internal conversion and intersystem crossing, which reduce the fluorescence quantum yield. The temperature dependence of fluorescence intensity can be described by the Arrhenius equation. However, in some cases, increasing temperature can lead to increased fluorescence intensity due to changes in the fluorophore’s conformation or aggregation state.
5.3 Solvent Polarity
The polarity of the solvent can affect the fluorescence intensity and emission wavelength of certain fluorophores. Polar solvents can stabilize the excited state of the fluorophore, leading to a red shift in the emission spectrum and a decrease in fluorescence intensity. Non-polar solvents can have the opposite effect, leading to a blue shift in the emission spectrum and an increase in fluorescence intensity. The effect of solvent polarity on fluorescence is known as solvatochromism. Solvatochromic fluorophores can be used as probes to measure the polarity of the local environment.
5.4 Quenching
Quenching is the process by which the fluorescence intensity of a fluorophore is reduced by interactions with other molecules. Quenching can occur through several mechanisms, including collisional quenching, Förster resonance energy transfer (FRET), and electron transfer. Collisional quenching occurs when the fluorophore interacts with a quencher molecule during its excited state lifetime. The quencher molecule can accept energy from the fluorophore, leading to non-radiative decay. FRET is a distance-dependent process in which energy is transferred from a donor fluorophore to an acceptor fluorophore. Electron transfer involves the transfer of an electron from the fluorophore to a quencher molecule or vice versa. Quenching can be used to probe molecular interactions and distances.
Many thanks to our sponsor Esdebe who helped us prepare this research report.
6. Applications of Fluorophores
Fluorophores have a wide range of applications in bioimaging, diagnostics, theranostics, materials science, and environmental monitoring.
6.1 Bioimaging
Fluorophores are widely used in bioimaging to visualize cells, tissues, and organisms. Fluorescence microscopy, flow cytometry, and fluorescence-activated cell sorting (FACS) are common techniques that utilize fluorophores. Fluorophores can be used to label specific cellular components, such as proteins, DNA, and lipids, allowing for the visualization of their localization, dynamics, and interactions. Fluorescent proteins, such as GFP and its derivatives, are particularly useful for bioimaging because they can be genetically encoded and expressed in living cells. Quantum dots offer exceptional brightness and photostability, making them ideal for long-term tracking studies and multiplexed imaging.
6.2 Diagnostics
Fluorophores are used in diagnostics to detect and quantify specific biomarkers in biological samples. Fluorescence immunoassays, such as ELISA (enzyme-linked immunosorbent assay), are widely used to detect and quantify antibodies, antigens, and other proteins. Fluorescence in situ hybridization (FISH) is used to detect and localize specific DNA sequences in cells and tissues. Fluorophores can also be used to detect and image tumors using techniques such as fluorescence endoscopy and fluorescence-guided surgery.
6.3 Theranostics
Theranostics is an emerging field that combines diagnostics and therapeutics. Fluorophores can be used to both image and treat diseases. For example, fluorophores can be conjugated to drugs or nanoparticles for targeted drug delivery. The fluorophore allows for the visualization of the drug delivery process, while the drug provides the therapeutic effect. Photodynamic therapy (PDT) is another example of theranostics that utilizes fluorophores. In PDT, a photosensitizer molecule, which is a type of fluorophore, is used to generate reactive oxygen species (ROS) upon exposure to light. The ROS kill cancer cells, providing a therapeutic effect.
6.4 Materials Science
Fluorophores are used in materials science to create fluorescent materials with various applications. Fluorescent polymers can be used in displays, sensors, and security inks. Fluorescent nanoparticles can be used in imaging and drug delivery. Fluorescent dyes can be used to label and track polymers and other materials.
6.5 Environmental Monitoring
Fluorophores are used in environmental monitoring to detect and quantify pollutants in water, air, and soil. Fluorescent sensors can be used to detect heavy metals, pesticides, and other contaminants. Fluorescent dyes can be used to track the movement of water and air.
Many thanks to our sponsor Esdebe who helped us prepare this research report.
7. Limitations and Challenges
Despite their widespread use, fluorophores have several limitations and challenges that need to be addressed.
7.1 Photobleaching
Photobleaching is the irreversible destruction of a fluorophore’s ability to fluoresce due to prolonged exposure to light. Photobleaching can limit the duration of imaging experiments and can affect the accuracy of quantitative measurements. Several strategies can be used to minimize photobleaching, including using more photostable fluorophores, reducing the excitation light intensity, and using antifade reagents.
7.2 Toxicity
Some fluorophores, particularly quantum dots containing cadmium, can be toxic to cells and organisms. The toxicity of fluorophores can limit their use in in vivo imaging and therapeutic applications. Efforts are underway to develop less toxic fluorophores based on alternative materials and to encapsulate fluorophores in biocompatible materials to reduce their toxicity.
7.3 Background Fluorescence
Background fluorescence can interfere with the detection of specific signals. Background fluorescence can be caused by autofluorescence from biological samples or by non-specific binding of fluorophores to unwanted targets. Several techniques can be used to reduce background fluorescence, including using fluorophores with large Stokes shifts, using time-resolved fluorescence imaging, and using blocking agents to reduce non-specific binding.
7.4 Spectral Overlap
The emission spectra of different fluorophores can overlap, making it difficult to distinguish between them in multicolor imaging experiments. Spectral overlap can be minimized by using fluorophores with narrow emission spectra and by using spectral unmixing techniques.
Many thanks to our sponsor Esdebe who helped us prepare this research report.
8. Future Directions
The field of fluorophore research is constantly evolving, with ongoing efforts to develop novel fluorophores with improved properties and expanded applications.
8.1 Stimuli-Responsive Fluorophores
Stimuli-responsive fluorophores are fluorophores that change their fluorescence properties in response to specific stimuli, such as pH, temperature, light, or the presence of specific molecules. These fluorophores can be used as sensors to monitor changes in the local environment. For example, pH-sensitive fluorophores can be used to measure pH changes in cells and tissues, while temperature-sensitive fluorophores can be used to measure temperature gradients.
8.2 Near-Infrared Emitting Fluorophores
Near-infrared (NIR) emitting fluorophores are fluorophores that emit light in the near-infrared region of the spectrum (700-900 nm). NIR light penetrates tissues more deeply than visible light, making NIR fluorophores ideal for in vivo imaging. NIR fluorophores can be used to image tumors, organs, and other tissues in living animals.
8.3 Biocompatible Fluorophores
Biocompatible fluorophores are fluorophores that are non-toxic and do not interfere with biological processes. These fluorophores are essential for in vivo imaging and therapeutic applications. Efforts are underway to develop biocompatible fluorophores based on organic dyes, fluorescent proteins, and quantum dots.
8.4 Fluorophores for Advanced Microscopy Techniques
Advanced microscopy techniques, such as super-resolution microscopy and light-sheet microscopy, require fluorophores with specific properties. Super-resolution microscopy requires fluorophores that can be switched on and off, while light-sheet microscopy requires fluorophores that are highly photostable. Efforts are underway to develop fluorophores that meet the requirements of these advanced microscopy techniques.
Many thanks to our sponsor Esdebe who helped us prepare this research report.
9. Conclusion
Fluorophores are versatile tools with a wide range of applications in various scientific disciplines. Their ability to emit light upon excitation has made them indispensable for bioimaging, diagnostics, materials science, and environmental monitoring. Continuous advancements in fluorophore research have led to the development of novel fluorophores with improved properties, such as enhanced brightness, photostability, biocompatibility, and spectral tunability. The integration of fluorophores with nanomaterials has further expanded their capabilities, enabling targeted delivery and enhanced imaging sensitivity. Despite their limitations, such as photobleaching and toxicity, fluorophores remain essential tools for scientific research and technological innovation. Future research will focus on developing stimuli-responsive fluorophores, near-infrared emitting fluorophores, and biocompatible fluorophores for advanced biological and biomedical applications. A deeper understanding of the photophysical properties and mechanisms of fluorescence will pave the way for the design of novel fluorophores with tailored properties for specific applications, further solidifying their role as indispensable tools in scientific endeavors.
Many thanks to our sponsor Esdebe who helped us prepare this research report.
References
- Lakowicz, J. R. (2006). Principles of Fluorescence Spectroscopy. Springer.
- Valeur, B., & Berberan-Santos, M. N. (2013). Molecular Fluorescence: Principles and Applications. Wiley-VCH.
- Resch-Genger, U., et al. (2008). Quantum Yields in Fluorescence Spectroscopy. Analytical and Bioanalytical Chemistry, 391(1), 1-21.
- Lichtman, J. W., & Conchello, J. A. (2005). Fluorescence Microscopy. Nature Methods, 2(12), 910-919.
- Tsien, R. Y. (1998). The Green Fluorescent Protein. Annual Review of Biochemistry, 67(1), 509-544.
- Michalet, X., et al. (2005). Quantum Dots for Live Cells, in Vivo Imaging, and Diagnostics. Science, 307(5709), 538-544.
- Bünzli, J. C. G. (2010). Lanthanide Luminescence for Biomedical Analyses and Imaging. Coordination Chemistry Reviews, 254(19-20), 1947-1962.
- Hildebrandt, N., & Algar, W. R. (2017). Quantum Dots as Donors in Förster Resonance Energy Transfer (FRET) Assays. Chemical Society Reviews, 46(15), 4224-4239.
- Waggoner, A. (2006). Fluorescent Labels for Combinatorial Chemistry and HTS. Current Opinion in Chemical Biology, 10(4), 303-309.
- Smith, A. M., & Nie, S. (2010). Quantum Dot-Based In Vivo Imaging. Accounts of Chemical Research, 43(2), 190-200.
- Frangioni, J. V. (2003). In Vivo Imaging and Genomics. Current Opinion in Biotechnology, 14(6), 618-627.
- Klar, T. A., & Hell, S. W. (1999). Subdiffraction Resolution in Far-Field Fluorescence Microscopy. Optics Letters, 24(14), 954-956.
- Pawley, J. B. (Ed.). (2006). Handbook of Biological Confocal Microscopy. Springer.
- Diaspro, A. (Ed.). (2010). Nanoscopy and Multidimensional Optical Imaging. CRC Press.
- Azzazy, H. M. E., Highsmith, W. E., & Christenson, R. H. (2002). Fluorescence Polarization Assays in Clinical Chemistry. Clinical Chemistry, 48(7), 1070-1087.
- Berezin, M. Y., & Achilefu, S. (2010). Fluorescence Lifetime Measurements and Biological Imaging. Chemical Reviews, 110(5), 2641-2684.
- Peng, X., et al. (2018). Recent Advances in Stimuli-Responsive Fluorescent Materials for Sensing and Imaging Applications. Chemical Society Reviews, 47(16), 6126-6151.
- Weissleder, R. (2001). Scaling Down Imaging: Molecular Sensors for MRI and Optical Imaging. Chemical Reviews, 101(10), 3175-3226.
- Yuan, H., et al. (2013). Near-Infrared II Biocompatible Luminescent Nanomaterials for In Vivo High-Contrast Imaging and Image-Guided Cancer Therapy. Advanced Materials, 25(43), 6070-6075.
- Chen, W., et al. (2016). Recent Advances in Biocompatible Quantum Dots for In Vivo Imaging. Journal of Materials Chemistry B, 4(12), 2069-2086.
The discussion of quenching phenomena and its impact on fluorescence intensity is quite interesting. Could the principles of quenching be adapted to develop more sensitive and selective biosensors, perhaps by designing molecules that exhibit a significant change in fluorescence upon binding to a specific target analyte?
That’s a brilliant point! The sensitivity and selectivity you describe could be a game-changer. By cleverly exploiting quenching and designing specific binding interactions, we could potentially create a new generation of biosensors with enhanced capabilities. Thanks for sparking such a valuable discussion!
Editor: MedTechNews.Uk
Thank you to our Sponsor Esdebe
The discussion of integrating fluorophores with nanoclays for targeted delivery is particularly exciting. Has there been exploration of using specific clay mineral types to control the release of fluorophores at the target site, enhancing both imaging and therapeutic efficacy?