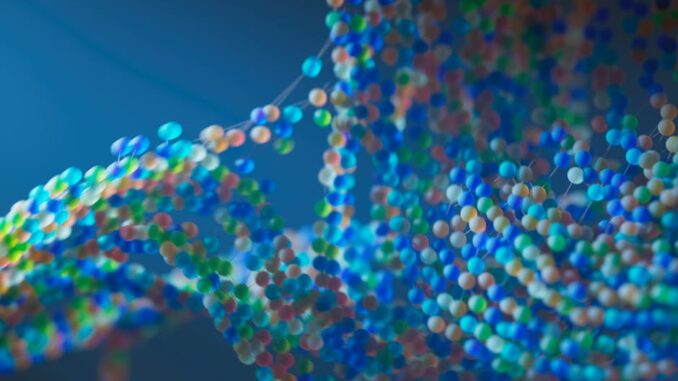
Abstract
Fluorescence imaging has emerged as a powerful and versatile technique in biological and biomedical research, offering high sensitivity, specificity, and the ability to visualize dynamic processes in real-time. This report provides a comprehensive overview of advanced fluorescence imaging, covering its fundamental principles, the diverse range of fluorescent probes available, and its widespread applications across various fields. We delve into the technological advancements that have pushed the boundaries of fluorescence imaging, including multi-photon microscopy, light-sheet microscopy, super-resolution microscopy, and fluorescence lifetime imaging microscopy (FLIM). Furthermore, we discuss the challenges associated with fluorescence imaging, such as photobleaching, autofluorescence, and probe toxicity, and explore strategies to mitigate these issues. Finally, we examine the future directions of fluorescence imaging, highlighting its potential to revolutionize our understanding of complex biological systems and improve diagnostic and therapeutic strategies.
Many thanks to our sponsor Esdebe who helped us prepare this research report.
1. Introduction
Fluorescence imaging is a non-destructive optical imaging technique that utilizes fluorescent molecules, known as fluorophores, to visualize specific structures or processes within a sample. The fundamental principle involves exciting a fluorophore with light at a specific wavelength, causing it to absorb energy and transition to an excited electronic state. Subsequently, the fluorophore returns to its ground state, emitting light at a longer wavelength, which is detected by a sensitive detector. This emitted light provides information about the location, concentration, and environment of the fluorophore, enabling researchers to study a wide range of biological phenomena with high spatiotemporal resolution.
The applications of fluorescence imaging are vast and continue to expand. It is widely used in cell biology to study protein localization, trafficking, and interactions; in neuroscience to visualize neuronal activity and connectivity; in immunology to track immune cell responses; and in cancer research to detect and monitor tumor growth and metastasis. Moreover, fluorescence imaging plays a crucial role in drug discovery, enabling the screening of potential therapeutic compounds and the assessment of their efficacy and toxicity.
While conventional fluorescence microscopy has proven invaluable, it is limited by factors such as diffraction, photobleaching, and autofluorescence. To overcome these limitations, researchers have developed advanced fluorescence imaging techniques that offer improved resolution, sensitivity, and penetration depth. These techniques, including multi-photon microscopy, light-sheet microscopy, and super-resolution microscopy, have revolutionized the field, enabling researchers to visualize subcellular structures and processes with unprecedented detail. This review aims to provide a broad overview of these advances.
Many thanks to our sponsor Esdebe who helped us prepare this research report.
2. Fundamentals of Fluorescence Imaging
At its core, fluorescence imaging hinges on the interaction of light with fluorescent molecules. When a fluorophore absorbs a photon of light at its excitation wavelength, an electron transitions to a higher energy state. This excited state is unstable, and the molecule rapidly returns to its ground state through various pathways, including vibrational relaxation, internal conversion, and fluorescence emission. Fluorescence emission involves the release of a photon with a longer wavelength (lower energy) than the excitation light, a phenomenon known as the Stokes shift. The magnitude of the Stokes shift is a characteristic property of each fluorophore and is crucial for separating the excitation and emission signals, allowing for selective detection of the emitted light.
The quantum yield of a fluorophore, defined as the ratio of emitted photons to absorbed photons, determines its brightness and is a key factor in determining its suitability for imaging applications. A high quantum yield indicates that the fluorophore is highly efficient at converting absorbed light into emitted light, resulting in a brighter signal and improved sensitivity.
The excitation and emission spectra of a fluorophore are also critical parameters that dictate its performance in fluorescence imaging. The excitation spectrum describes the efficiency of light absorption as a function of wavelength, while the emission spectrum describes the intensity of emitted light as a function of wavelength. By carefully selecting fluorophores with distinct excitation and emission spectra, researchers can simultaneously image multiple targets in a single sample, a technique known as multi-color imaging.
Many thanks to our sponsor Esdebe who helped us prepare this research report.
3. Fluorescent Probes: A Diverse Toolkit
A wide variety of fluorescent probes are available for fluorescence imaging, each with unique properties and applications. These probes can be broadly classified into organic dyes, fluorescent proteins, quantum dots, and genetically encoded fluorescent indicators.
3.1 Organic Dyes
Organic dyes are small synthetic molecules that exhibit strong fluorescence and can be easily conjugated to antibodies, peptides, and other biomolecules. Common examples include Alexa Fluor dyes, Cy dyes, and rhodamine dyes. These dyes offer high brightness, good photostability, and a wide range of excitation and emission wavelengths, making them versatile tools for labeling and imaging various cellular structures and processes. However, organic dyes can suffer from drawbacks such as limited cell permeability and potential toxicity.
3.2 Fluorescent Proteins
Fluorescent proteins (FPs), such as green fluorescent protein (GFP) and its variants, are genetically encoded fluorescent markers that can be expressed within living cells. FPs revolutionized fluorescence imaging by allowing researchers to directly visualize gene expression, protein localization, and protein-protein interactions in real-time. FPs offer advantages such as biocompatibility and the ability to target specific cellular compartments. However, FPs can be less bright than organic dyes and may exhibit photobleaching.
3.3 Quantum Dots
Quantum dots (QDs) are semiconductor nanocrystals that exhibit unique optical properties, including broad excitation spectra, narrow emission spectra, and high brightness. QDs are highly photostable and can be conjugated to biomolecules for targeted imaging. They are particularly useful for multi-color imaging and long-term tracking studies. However, QDs can be toxic due to their heavy metal composition and may exhibit blinking, a phenomenon where their fluorescence intensity fluctuates over time.
3.4 Genetically Encoded Fluorescent Indicators
Genetically encoded fluorescent indicators (GEFIs) are fluorescent proteins that are engineered to respond to specific cellular signals, such as calcium, pH, or voltage. GEFIs allow researchers to monitor dynamic changes in these signals in real-time. For example, calcium indicators like GCaMP are widely used to study neuronal activity and calcium signaling in various cell types. GEFIs provide a powerful tool for studying cellular physiology and disease.
The development of new and improved fluorescent probes remains an active area of research. Researchers are continuously developing probes with improved brightness, photostability, biocompatibility, and targeting specificity.
Many thanks to our sponsor Esdebe who helped us prepare this research report.
4. Advanced Fluorescence Imaging Techniques
To overcome the limitations of conventional fluorescence microscopy, researchers have developed a range of advanced imaging techniques that offer improved resolution, sensitivity, and penetration depth.
4.1 Confocal Microscopy
Confocal microscopy uses a pinhole aperture to block out-of-focus light, resulting in sharper images and improved resolution. By scanning the sample in three dimensions, confocal microscopy can generate optical sections that can be reconstructed into a 3D image. Confocal microscopy is widely used to study cellular structures and processes in thick samples.
4.2 Multi-Photon Microscopy
Multi-photon microscopy uses pulsed lasers to excite fluorophores with two or more photons simultaneously. This technique offers several advantages over conventional fluorescence microscopy, including deeper penetration depth, reduced photobleaching, and reduced photodamage. Multi-photon microscopy is particularly useful for imaging live tissues and organisms.
4.3 Light-Sheet Microscopy
Light-sheet microscopy, also known as selective plane illumination microscopy (SPIM), uses a thin sheet of light to illuminate the sample, minimizing photobleaching and photodamage. This technique allows for rapid 3D imaging of live samples with high resolution. Light-sheet microscopy is particularly useful for imaging developing embryos and other large, transparent samples.
4.4 Super-Resolution Microscopy
Super-resolution microscopy techniques overcome the diffraction limit of light, allowing researchers to visualize structures and processes with unprecedented resolution. Several super-resolution techniques have been developed, including stimulated emission depletion (STED) microscopy, structured illumination microscopy (SIM), and single-molecule localization microscopy (SMLM). STED microscopy uses a depletion laser to narrow the fluorescence emission area, resulting in improved resolution. SIM uses structured illumination patterns to extract high-resolution information from the sample. SMLM techniques, such as photoactivated localization microscopy (PALM) and stochastic optical reconstruction microscopy (STORM), rely on the stochastic activation and localization of individual fluorophores to reconstruct a high-resolution image. Super-resolution microscopy has revolutionized cell biology, allowing researchers to visualize subcellular structures and processes with nanometer-scale resolution.
4.5 Fluorescence Lifetime Imaging Microscopy (FLIM)
FLIM measures the fluorescence lifetime of a fluorophore, which is the average time it spends in the excited state before returning to the ground state. Fluorescence lifetime is sensitive to the microenvironment of the fluorophore, including pH, temperature, and the presence of other molecules. FLIM can be used to study protein-protein interactions, enzyme activity, and other cellular processes. It is relatively insensitive to fluorophore concentration and photobleaching, making it a robust technique for quantitative imaging.
The choice of imaging technique depends on the specific research question and the characteristics of the sample. Researchers often combine multiple imaging techniques to obtain a comprehensive understanding of the system under study.
Many thanks to our sponsor Esdebe who helped us prepare this research report.
5. Applications of Fluorescence Imaging
Fluorescence imaging has become an indispensable tool in a wide range of scientific disciplines, including:
5.1 Cell Biology
Fluorescence imaging is widely used in cell biology to study protein localization, trafficking, and interactions. Researchers use fluorescently labeled antibodies, proteins, and small molecules to visualize specific cellular structures and processes. Fluorescence imaging is also used to study cell division, cell migration, and cell signaling.
5.2 Neuroscience
Fluorescence imaging plays a crucial role in neuroscience research. Researchers use fluorescent probes to monitor neuronal activity, visualize neuronal connectivity, and study synaptic transmission. Calcium imaging, a type of fluorescence imaging that uses calcium-sensitive dyes, is widely used to study neuronal activity in response to various stimuli. Fluorescence imaging is also used to study neurodegenerative diseases, such as Alzheimer’s disease and Parkinson’s disease.
5.3 Immunology
Fluorescence imaging is used to track immune cell responses, study immune cell interactions, and visualize the trafficking of immune cells in vivo. Researchers use fluorescently labeled antibodies to identify and track different types of immune cells. Fluorescence imaging is also used to study the immune response to infections and vaccines.
5.4 Cancer Research
Fluorescence imaging is widely used in cancer research to detect and monitor tumor growth and metastasis. Researchers use fluorescently labeled antibodies and small molecules to target specific cancer cells. Fluorescence imaging is also used to study the tumor microenvironment and the response of tumors to therapy. In the operating room, fluorescence guided surgery, where a tumour specific fluorescent dye highlights cancer cells allows surgeons to visualise otherwise unidentifiable cancer cells and improve resection accuracy.
5.5 Drug Discovery
Fluorescence imaging plays a crucial role in drug discovery by enabling the screening of potential therapeutic compounds and the assessment of their efficacy and toxicity. High-throughput screening platforms use fluorescence imaging to identify compounds that modulate specific cellular targets. Fluorescence imaging is also used to study the mechanism of action of drugs and to assess their effects on cellular processes.
Many thanks to our sponsor Esdebe who helped us prepare this research report.
6. Challenges and Limitations
Despite its versatility and power, fluorescence imaging is not without its challenges and limitations. Some of the major challenges include:
6.1 Photobleaching
Photobleaching is the irreversible destruction of a fluorophore due to prolonged exposure to excitation light. Photobleaching reduces the fluorescence signal over time and can limit the duration of imaging experiments. To minimize photobleaching, researchers can use lower excitation light intensities, shorter exposure times, and anti-fade reagents.
6.2 Autofluorescence
Autofluorescence is the emission of light from endogenous molecules within the sample. Autofluorescence can interfere with the fluorescence signal from the probe of interest and reduce the signal-to-noise ratio. To minimize autofluorescence, researchers can use longer excitation wavelengths, spectral unmixing techniques, and genetically encoded fluorescent probes.
6.3 Probe Toxicity
Some fluorescent probes can be toxic to cells, particularly at high concentrations. Probe toxicity can interfere with cellular processes and lead to inaccurate results. To minimize probe toxicity, researchers can use lower probe concentrations, biocompatible probes, and delivery methods that target the probe to specific cellular compartments.
6.4 Light Scattering and Absorption
In thick tissues, light scattering and absorption can limit the penetration depth of excitation and emission light. This can result in reduced signal intensity and image quality. To overcome this limitation, researchers can use longer excitation wavelengths, optical clearing techniques, and multi-photon microscopy.
Many thanks to our sponsor Esdebe who helped us prepare this research report.
7. Future Directions and Emerging Trends
The field of fluorescence imaging is constantly evolving, with new technologies and applications emerging at a rapid pace. Some of the key future directions and emerging trends include:
7.1 Development of New Fluorescent Probes
Researchers are continuously developing new fluorescent probes with improved brightness, photostability, biocompatibility, and targeting specificity. This includes the development of probes that respond to specific cellular signals, such as reactive oxygen species, proteases, and metabolites. The rational design of novel fluorophores using computational chemistry is also accelerating probe development.
7.2 Integration with Artificial Intelligence
Artificial intelligence (AI) is being increasingly used to analyze and interpret fluorescence imaging data. AI algorithms can be used to automatically segment cells, track cellular movements, and quantify fluorescence signals. AI can also be used to correct for image artifacts and to improve the signal-to-noise ratio. Deep learning, a type of AI, is particularly well-suited for analyzing complex fluorescence imaging datasets.
7.3 Advanced Microscopy Techniques
New and improved microscopy techniques are being developed to push the boundaries of fluorescence imaging. This includes the development of faster and more sensitive detectors, improved optical designs, and new methods for data analysis. Adaptive optics, a technique used to correct for aberrations in the optical path, is being increasingly used in fluorescence microscopy to improve image quality.
7.4 Expansion into Clinical Applications
Fluorescence imaging is being increasingly used in clinical applications, such as diagnosis, image-guided surgery, and drug delivery. For example, fluorescence-guided surgery is being used to improve the accuracy of tumor resection. Fluorescence imaging is also being used to monitor the response of patients to therapy. The development of new and improved fluorescent probes that are safe and effective for use in humans is crucial for the widespread adoption of fluorescence imaging in clinical settings. Furthermore, portable and point-of-care fluorescence imaging devices are being developed to enable rapid and convenient diagnostics.
7.5 Multimodal Imaging
Combining fluorescence imaging with other imaging modalities, such as magnetic resonance imaging (MRI), computed tomography (CT), and positron emission tomography (PET), can provide a more comprehensive understanding of complex biological systems. Multimodal imaging allows researchers to correlate structural, functional, and molecular information, leading to a more complete picture of the system under study.
Many thanks to our sponsor Esdebe who helped us prepare this research report.
8. Conclusion
Fluorescence imaging has revolutionized biological and biomedical research, providing a powerful tool for visualizing and studying complex biological systems. The continuous development of new fluorescent probes, advanced imaging techniques, and computational tools is driving the field forward. Future directions include the integration of AI, the development of multimodal imaging approaches, and the expansion of fluorescence imaging into clinical applications. As technology advances, fluorescence imaging will continue to play a central role in advancing our understanding of biology and improving human health.
Many thanks to our sponsor Esdebe who helped us prepare this research report.
References
- Lakowicz, J. R. (2006). Principles of fluorescence spectroscopy. Springer.
- Lichtman, J. W., & Conchello, J. A. (2005). Fluorescence microscopy. Nature Methods, 2(12), 910-919.
- Helmchen, F., & Denk, W. (2005). Deep tissue two-photon microscopy. Nature Methods, 2(12), 932-940.
- Sauer, M., & Heilemann, M. (2017). Super-resolution microscopy. Chemical Reviews, 117(12), 8026-8063.
- Diaspro, A., Castello, M., Vicidomini, G., & Faretta, M. (2016). Structured illumination microscopy (SIM) and super-resolved techniques. Journal of Biophotonics, 9(10), 1064-1075.
- Shaner, N. C., Steinbach, P. A., & Tsien, R. Y. (2005). Improving the photostability of bright monomeric fluorescent proteins. Nature Biotechnology, 23(12), 1557-1560.
- Pawley, J. B. (Ed.). (2006). Handbook of biological confocal microscopy. Springer.
- Zipfel, W. R., Williams, R. M., Christie, R., Colbert, D. L., & Webb, W. W. (2003). Live tissue intrinsic emission microscopy using multiphoton-excited native fluorescence. Proceedings of the National Academy of Sciences, 100(12), 7075-7080.
- Stelzer, E. H. K. (2015). Light-sheet microscopy for live sciences. Nature Methods, 12(1), 23-26.
- Gustafsson, M. G. (2005). Nonlinear structured-illumination microscopy: wide-field fluorescence imaging with theoretically unlimited resolution. Proceedings of the National Academy of Sciences, 102(37), 13081-13086.
- Betzig, E., Patterson, G. H., Sougrat, R., Lindwasser, O. W., Olenych, S., Bonifacino, J. S., … & Lippincott-Schwartz, J. (2006). Imaging intracellular fluorescent proteins at nanometer resolution. Science, 313(5793), 1642-1645.
- Rust, M. J., Bates, M., & Zhuang, X. (2006). Sub-diffraction-limit imaging by stochastic optical reconstruction microscopy (STORM). Nature Methods, 3(10), 793-795.
- Becker, W. (2012). Advanced time-correlated single photon counting methods. Springer.
- Berezin, M. Y., & Achilefu, S. (2010). Fluorescence lifetime imaging microscopy (FLIM): instrumentation and medical applications. Chemical Reviews, 110(5), 2641-2684.
- Kircher, M. F., Willmann, J. K., Goumas, M. T., Serkova, N. J., & Gambhir, S. S. (2012). Image-guided cancer surgery using near-infrared fluorescent dye. Cancer Research, 72(21), 5559-5569.
- Ntziachristos, V. (2010). Fluorescence molecular imaging. Annual Review of Biomedical Engineering, 12, 333-368.
- Frangioni, J. V. (2003). In vivo imaging with quantum dots. Current Opinion in Chemical Biology, 7(5), 626-634.
- Giepmans, B. N., Adams, S. R., Ellisman, M. H., & Tsien, R. Y. (2006). The fluorescent toolbox for assessing protein location and function. Science, 312(5771), 217-224.
- Miller, E. W., Lin, J. Y., Frady, E. P., Steinbach, P. A., & Tsien, R. Y. (2005). Fluorescent যাক-labels to study cellular dynamics. ChemBioChem, 6(7), 1107-1119.
- Weissleder, R. (2001). Scaling down imaging: molecular sensors for cells. Nature Biotechnology, 19(4), 316-317.
The overview of advanced microscopy techniques, particularly super-resolution microscopy, is compelling. The ability to visualize cellular structures at the nanometer scale opens exciting avenues for understanding disease mechanisms and developing targeted therapies.
Thanks for highlighting the section on super-resolution microscopy! It’s exciting to think about how visualizing things at that scale will change how we approach treatments and diagnostics. What applications are you most eager to see developed using this technology?
Editor: MedTechNews.Uk
Thank you to our Sponsor Esdebe
Fascinating review! With all these advances in fluorescence imaging, are we nearing a point where cells are going to feel like they’re constantly under surveillance, and will they start developing countermeasures? Like, maybe evolving super-autofluorescence as a cloaking device?
That’s a truly fascinating thought! The idea of cells evolving ‘cloaking devices’ in response to advanced imaging is very creative. It highlights the dynamic interaction between our observation methods and the biological systems we study. Perhaps future research will reveal novel cellular mechanisms developed as a result of these selective pressures. Thank you for your comment!
Editor: MedTechNews.Uk
Thank you to our Sponsor Esdebe
The overview of future directions is insightful, especially the integration of AI for image analysis. How might AI-driven tools further minimize photobleaching or autofluorescence during live-cell imaging, perhaps through real-time parameter adjustments?
That’s a great question! AI could analyze the rate of photobleaching in real-time and adjust laser intensity or exposure time dynamically to minimize damage. Similarly, AI could identify and subtract autofluorescence signatures, enhancing image clarity without compromising cell viability. This adaptive approach offers exciting possibilities for gentle, long-term live-cell imaging.
Editor: MedTechNews.Uk
Thank you to our Sponsor Esdebe
AI stepping in to analyze images? Soon we’ll have microscopes complaining about their algorithms being biased against blurry cells. “They’re just misunderstood, not out of focus!”