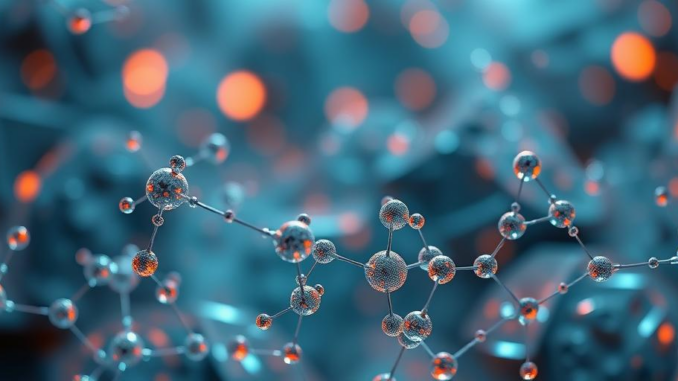
Abstract
Nanoplatforms, engineered nanomaterials with multifaceted functionalities, have emerged as a promising avenue in modern medicine, particularly in cancer therapy. This report delves into the diverse landscape of nanoplatform technologies, examining their design principles, synthetic methodologies, surface functionalization strategies, delivery mechanisms, targeting capabilities, biocompatibility considerations, and safety profiles. Beyond cancer, the report explores the application of nanoplatforms in other therapeutic areas, including infectious disease treatment, regenerative medicine, and diagnostics. We critically assess the challenges associated with clinical translation, focusing on scalability, reproducibility, and regulatory hurdles. Furthermore, we provide an outlook on the future directions of nanoplatform research, highlighting the integration of artificial intelligence, personalized medicine approaches, and advanced imaging techniques to enhance their efficacy and broaden their applicability.
Many thanks to our sponsor Esdebe who helped us prepare this research report.
1. Introduction
The convergence of nanotechnology and medicine has revolutionized therapeutic and diagnostic approaches, giving rise to the field of nanomedicine. At the heart of nanomedicine lies the concept of nanoplatforms – meticulously engineered nanomaterials designed to perform specific functions at the nanoscale. These platforms, ranging in size from 1 to 1000 nanometers, offer several advantages over conventional therapies, including enhanced drug delivery, targeted action, reduced systemic toxicity, and improved diagnostic capabilities.
Traditional treatment modalities for diseases like cancer often suffer from limitations such as non-specific targeting, high systemic toxicity, and poor bioavailability. Nanoplatforms address these limitations by providing a versatile framework for encapsulating, delivering, and releasing therapeutic agents directly to the diseased site. Moreover, nanoplatforms can be functionalized with targeting ligands, enabling selective binding to specific cells or tissues. Their small size allows them to passively accumulate in tumors through the enhanced permeability and retention (EPR) effect, a phenomenon characterized by leaky tumor vasculature and impaired lymphatic drainage.
This report provides a comprehensive overview of nanoplatform technologies, examining their design principles, synthesis methods, functionalization strategies, delivery mechanisms, biocompatibility considerations, and clinical translation challenges. It also explores the application of nanoplatforms beyond cancer, highlighting their potential in treating other diseases and improving diagnostic capabilities. Furthermore, the report discusses the future directions of nanoplatform research, emphasizing the importance of personalized medicine and advanced imaging techniques.
Many thanks to our sponsor Esdebe who helped us prepare this research report.
2. Design Principles and Material Selection
The design of effective nanoplatforms is a multifaceted process that requires careful consideration of several factors, including material selection, size, shape, surface properties, and biodegradability. The choice of materials plays a critical role in determining the biocompatibility, stability, and functionality of the nanoplatform.
2.1 Material Types
- Liposomes: Composed of lipid bilayers, liposomes are biocompatible and biodegradable, making them ideal for encapsulating both hydrophilic and hydrophobic drugs. Their versatility allows for surface modification with targeting ligands and polyethylene glycol (PEG) to enhance circulation time. However, liposomes may suffer from instability and rapid drug leakage.
- Polymeric Nanoparticles: Synthetic polymers, such as poly(lactic-co-glycolic acid) (PLGA), polycaprolactone (PCL), and polyethyleneimine (PEI), are widely used for nanoplatform fabrication. PLGA is biodegradable and biocompatible, making it a popular choice for drug delivery. PCL offers slower degradation rates, while PEI exhibits high transfection efficiency for gene therapy applications. However, the potential toxicity of certain polymers must be carefully evaluated.
- Inorganic Nanoparticles: Gold nanoparticles (AuNPs), silica nanoparticles (SiO2), and quantum dots (QDs) possess unique optical and electronic properties, making them attractive for imaging, sensing, and photothermal therapy. AuNPs exhibit strong surface plasmon resonance, which can be exploited for photothermal ablation of cancer cells. SiO2 nanoparticles are biocompatible and can be easily functionalized with various molecules. QDs are semiconductor nanocrystals that emit bright, size-tunable fluorescence, making them useful for high-resolution imaging. However, the potential toxicity and long-term fate of inorganic nanoparticles remain a concern.
- Carbon-Based Nanomaterials: Carbon nanotubes (CNTs) and graphene are carbon allotropes with exceptional mechanical strength, thermal conductivity, and electrical conductivity. CNTs can be functionalized with drugs, genes, and targeting ligands for targeted drug delivery. Graphene, a two-dimensional sheet of carbon atoms, exhibits high surface area and excellent drug loading capacity. However, the potential toxicity and biocompatibility of CNTs and graphene require further investigation.
- Metal-Organic Frameworks (MOFs): MOFs are crystalline materials composed of metal ions coordinated to organic ligands, forming porous structures with high surface area and tunable pore size. MOFs can be used to encapsulate large amounts of drugs and deliver them in a controlled manner. Their biodegradability and biocompatibility can be improved by using biocompatible metal ions and organic ligands.
2.2 Size and Shape
The size and shape of nanoplatforms influence their biodistribution, cellular uptake, and therapeutic efficacy. Smaller nanoparticles (10-100 nm) tend to exhibit better penetration into tumors and tissues, while larger nanoparticles (100-200 nm) may be more efficiently taken up by phagocytic cells. The shape of nanoplatforms also plays a role in their cellular uptake and biodistribution. Spherical nanoparticles are generally cleared more rapidly than rod-shaped or disc-shaped nanoparticles.
2.3 Surface Properties
The surface properties of nanoplatforms, such as charge, hydrophobicity, and surface chemistry, affect their interactions with biological systems. Positively charged nanoparticles tend to interact more strongly with negatively charged cell membranes, leading to enhanced cellular uptake. Hydrophilic nanoparticles exhibit longer circulation times in the bloodstream compared to hydrophobic nanoparticles. Surface modification with PEG can further enhance circulation time by reducing protein adsorption and opsonization.
Many thanks to our sponsor Esdebe who helped us prepare this research report.
3. Methods of Synthesis and Functionalization
The synthesis of nanoplatforms involves a variety of techniques, including self-assembly, microemulsion, nanoprecipitation, and layer-by-layer assembly. The choice of synthesis method depends on the desired material, size, shape, and surface properties of the nanoplatform.
3.1 Synthesis Methods
- Self-Assembly: Self-assembly involves the spontaneous organization of molecules into ordered structures. Liposomes, micelles, and polymersomes can be formed through self-assembly of lipids, surfactants, and amphiphilic polymers, respectively.
- Microemulsion: Microemulsions are thermodynamically stable dispersions of two immiscible liquids. They can be used to synthesize nanoparticles with controlled size and shape.
- Nanoprecipitation: Nanoprecipitation involves the rapid mixing of a polymer solution with a non-solvent, leading to the formation of nanoparticles.
- Layer-by-Layer Assembly: Layer-by-layer assembly involves the sequential deposition of oppositely charged polyelectrolytes onto a template, creating multilayered films or capsules.
3.2 Functionalization Strategies
Surface functionalization is essential for enhancing the targeting capabilities, biocompatibility, and therapeutic efficacy of nanoplatforms. Functionalization can be achieved through various methods, including chemical conjugation, physical adsorption, and encapsulation.
- Chemical Conjugation: Chemical conjugation involves the covalent attachment of molecules to the surface of nanoplatforms. This method allows for precise control over the type and density of molecules attached to the surface. Common conjugation chemistries include carbodiimide coupling, thiol-maleimide coupling, and click chemistry.
- Physical Adsorption: Physical adsorption involves the non-covalent binding of molecules to the surface of nanoplatforms through electrostatic interactions, van der Waals forces, or hydrophobic interactions. This method is simpler than chemical conjugation but may result in less stable surface modifications.
- Encapsulation: Encapsulation involves the entrapment of molecules within the nanoplatform. This method is useful for protecting drugs and genes from degradation and controlling their release.
Many thanks to our sponsor Esdebe who helped us prepare this research report.
4. Delivery Mechanisms and Targeting Strategies
Effective delivery of therapeutic agents to the diseased site is crucial for achieving optimal therapeutic outcomes. Nanoplatforms can be designed to deliver drugs through various mechanisms, including passive targeting, active targeting, and stimuli-responsive release.
4.1 Passive Targeting
Passive targeting relies on the inherent properties of nanoplatforms to accumulate in tumors through the enhanced permeability and retention (EPR) effect. The EPR effect is characterized by leaky tumor vasculature and impaired lymphatic drainage, which allows nanoparticles to preferentially accumulate in tumors.
4.2 Active Targeting
Active targeting involves the modification of nanoplatforms with targeting ligands that specifically bind to receptors overexpressed on cancer cells or other diseased cells. Common targeting ligands include antibodies, peptides, aptamers, and small molecules. Active targeting can enhance the selectivity and efficacy of nanoplatforms.
4.3 Stimuli-Responsive Release
Stimuli-responsive release involves the design of nanoplatforms that release their payload in response to specific stimuli present at the diseased site. These stimuli can include changes in pH, temperature, redox potential, or enzyme activity. Stimuli-responsive release can improve the therapeutic efficacy of nanoplatforms by ensuring that drugs are released only at the site of action.
Many thanks to our sponsor Esdebe who helped us prepare this research report.
5. Biocompatibility and Safety Profiles
Biocompatibility and safety are paramount concerns in the development of nanoplatforms for clinical applications. Nanoplatforms must be non-toxic, non-immunogenic, and biodegradable or excretable from the body.
5.1 Biocompatibility Assessment
Biocompatibility assessment involves evaluating the interactions of nanoplatforms with biological systems, including cells, tissues, and organs. In vitro studies are typically performed to assess the cytotoxicity, genotoxicity, and immunogenicity of nanoplatforms. In vivo studies are conducted to evaluate the biodistribution, toxicity, and long-term fate of nanoplatforms in animal models.
5.2 Factors Affecting Biocompatibility
Several factors can affect the biocompatibility of nanoplatforms, including material composition, size, shape, surface charge, and surface chemistry. Nanomaterials with high surface area, positive charge, and hydrophobic surface tend to be more toxic than nanomaterials with low surface area, negative charge, and hydrophilic surface.
5.3 Strategies for Improving Biocompatibility
Various strategies can be employed to improve the biocompatibility of nanoplatforms, including the use of biocompatible materials, surface modification with PEG, and encapsulation of toxic materials.
Many thanks to our sponsor Esdebe who helped us prepare this research report.
6. Clinical Translation Challenges and Current Status
Despite the significant progress in nanoplatform research, clinical translation remains a major challenge. Several hurdles must be overcome to bring nanoplatform-based therapies to the market, including scalability, reproducibility, regulatory issues, and cost-effectiveness.
6.1 Scalability and Reproducibility
The synthesis of nanoplatforms must be scalable and reproducible to meet the demands of clinical trials and commercial production. Batch-to-batch variability in size, shape, and surface properties can affect the efficacy and safety of nanoplatforms.
6.2 Regulatory Issues
The regulatory landscape for nanomedicines is complex and evolving. Regulatory agencies, such as the FDA in the United States and the EMA in Europe, require rigorous testing and characterization of nanoplatforms to ensure their safety and efficacy.
6.3 Cost-Effectiveness
The cost of manufacturing nanoplatforms can be high, which may limit their accessibility to patients. Efforts are needed to develop more cost-effective synthesis methods and manufacturing processes.
6.4 Current Status
Several nanoplatform-based therapies have been approved for clinical use, including liposomal doxorubicin (Doxil/Caelyx) for the treatment of ovarian cancer and multiple myeloma, albumin-bound paclitaxel (Abraxane) for the treatment of breast cancer, and iron oxide nanoparticles (Ferrofluid) for the treatment of iron deficiency anemia. However, the number of approved nanomedicines remains relatively small compared to the vast number of nanoplatforms under development.
Many thanks to our sponsor Esdebe who helped us prepare this research report.
7. Nanoplatforms Beyond Cancer: Expanding Therapeutic Horizons
While cancer therapy has been a primary focus, the versatility of nanoplatforms extends to a wide range of other therapeutic areas. This section explores their applications in infectious disease treatment, regenerative medicine, and diagnostics.
7.1 Infectious Disease Treatment
Nanoplatforms offer novel approaches to combatting infectious diseases. They can be used to:
- Deliver Antimicrobials: Encapsulating antibiotics, antivirals, or antifungals within nanocarriers enhances drug delivery to infected cells, reduces systemic toxicity, and overcomes drug resistance mechanisms. Specific targeting to infected tissues or cells can further improve therapeutic efficacy.
- Develop Vaccines: Nanoparticles can serve as vaccine delivery vehicles, enhancing antigen presentation and stimulating a robust immune response. Self-adjuvanting nanoparticles, which incorporate both antigen and adjuvant, offer a promising strategy for improved vaccine efficacy.
- Target Viral Entry: Nanoparticles can be designed to interfere with viral entry into cells, preventing infection. This approach is particularly relevant for viruses that utilize specific receptor-mediated mechanisms for cell entry.
7.2 Regenerative Medicine
Nanoplatforms play a crucial role in regenerative medicine by:
- Delivering Growth Factors and Stem Cells: Nanocarriers can deliver growth factors or stem cells to damaged tissues, promoting tissue regeneration and repair. Controlled release of growth factors can optimize cell proliferation and differentiation.
- Creating Scaffolds for Tissue Engineering: Nanomaterials can be used to create scaffolds that mimic the extracellular matrix, providing a supportive environment for cell attachment and tissue formation. The mechanical and biological properties of the scaffolds can be tailored to specific tissue requirements.
- Monitoring Tissue Regeneration: Nanoparticles can be used as imaging agents to monitor tissue regeneration in real-time, providing valuable information about the effectiveness of regenerative therapies.
7.3 Diagnostics
Nanoplatforms have revolutionized diagnostic imaging and sensing by:
- Enhancing Contrast in Imaging Modalities: Nanoparticles can be used as contrast agents in various imaging modalities, such as MRI, CT, and ultrasound, enhancing the visibility of tumors, lesions, and other abnormalities. Targeted nanoparticles can provide highly specific and sensitive imaging.
- Developing Biosensors for Disease Detection: Nanomaterials can be integrated into biosensors for the rapid and accurate detection of biomarkers associated with various diseases. These biosensors can be used for point-of-care diagnostics, enabling early detection and treatment.
- Facilitating Theranostics: Theranostics combines diagnostics and therapeutics into a single platform, allowing for personalized treatment strategies. Nanoplatforms can be designed to both diagnose and treat diseases, optimizing therapeutic outcomes.
Many thanks to our sponsor Esdebe who helped us prepare this research report.
8. Future Directions and Conclusion
The field of nanoplatform research is rapidly evolving, with new materials, synthesis methods, and functionalization strategies constantly being developed. Future research will focus on several key areas:
- Personalized Medicine: Tailoring nanoplatforms to individual patients based on their genetic makeup, disease stage, and treatment history will improve therapeutic efficacy and reduce side effects. This requires advanced diagnostic tools to identify biomarkers and predict treatment response.
- Artificial Intelligence (AI): AI can be used to optimize the design of nanoplatforms, predict their behavior in vivo, and analyze large datasets to identify new therapeutic targets. Machine learning algorithms can accelerate the development of new nanomedicines.
- Advanced Imaging Techniques: Integrating advanced imaging techniques, such as intravital microscopy and multi-photon microscopy, will allow for real-time monitoring of nanoplatform biodistribution, cellular uptake, and drug release. This information can be used to optimize nanoplatform design and delivery strategies.
- Improved Biocompatibility and Safety: Further research is needed to improve the biocompatibility and safety of nanoplatforms, particularly for long-term applications. This includes developing biodegradable and excretable nanomaterials, as well as understanding the potential long-term effects of nanomaterials on the body.
- Scale-up and Manufacturing: Developing scalable and cost-effective manufacturing processes is essential for the widespread adoption of nanoplatform-based therapies. This requires collaboration between researchers, engineers, and industry partners.
In conclusion, nanoplatforms offer a versatile and promising approach to treating a wide range of diseases. While challenges remain, ongoing research and technological advancements are paving the way for the development of more effective, safer, and personalized nanomedicines. The integration of AI, advanced imaging techniques, and personalized medicine approaches will further accelerate the translation of nanoplatforms into clinical practice, ultimately improving patient outcomes.
Many thanks to our sponsor Esdebe who helped us prepare this research report.
References
- Ferrari, M. (2005). Cancer nanotechnology: opportunities and challenges. Nature Reviews Cancer, 5(3), 161-171.
- Peer, D., Karp, J. M., Hong, S., FaroKhzad, O. C., Margalit, R., & Langer, R. (2007). Nanocarriers for targeted cancer therapy. Nature Nanotechnology, 2(12), 751-760.
- Davis, M. E., Zuckerman, J. E., Choi, C. H. J., Shin, Y. K., Cullis, P. R., Ansari, D., … & Chen, I. W. (2010). Evidence of RNAi in humans from systemically administered siRNA via targeted nanoparticles. Nature, 464(7291), 1067-1070.
- Barreto, J. A., O’Malley, W., Kubeil, M., Graham, B., Stephanopoulos, N., Heinz, H., & Rotello, V. M. (2011). Quantum dots for combined detection and therapy. Advanced Materials, 23(18), H18-H40.
- Blanco, E., Shen, H., & Ferrari, M. (2015). Principles of nanoparticle design for overcoming biological barriers to drug delivery. Nature Biotechnology, 33(9), 941-951.
- Mura, S., Nicolas, J., & Couvreur, P. (2013). Stimuli-responsive nanocarriers for drug delivery. Nature Materials, 12(11), 991-1003.
- Allen, T. M., & Cullis, P. R. (2004). Drug delivery systems: entering the mainstream. Science, 303(5665), 1818-1822.
- Danhier, F., Feron, O., Preat, V., & Langer, R. (2010). To exploit the tumor microenvironment: passive and active tumor targeting strategies for anticancer nanomedicines. Journal of Controlled Release, 148(2), 135-146.
- Wilhelm, S., Tavares, A. J., Gazeau, F., Roch, A., Zhang, C., Pellegrino, T., & Chan, W. C. W. (2016). Analysis of nanoparticle delivery to tumours. Nature Reviews Materials, 1(5), 1-12.
- Wicki, A., Witzigmann, D., Balasubramanian, V., & Huwyler, J. (2015). Nanomedicine in cancer therapy: past, present and future. Pharmaceutical Research, 32(9), 2925-2946.
- Ventola, C. L. (2015). The antibiotic resistance crisis: part 1: causes and threats. Pharmacy and Therapeutics, 40(4), 277.
- Anselmo, A. C., & Mitragotri, S. (2019). Nanoparticles in vaccines. Wiley Interdisciplinary Reviews: Nanomedicine and Nanobiotechnology, 11(1), e1533.
- Drury, J. L., & Mooney, D. J. (2003). Hydrogels for tissue engineering: scaffold design variables and applications. Biomaterials, 24(24), 4337-4351.
- Kim, D., Lee, N., Park, M., Shin, T. H., An, K., & Hyeon, T. (2011). Synthesis of uniform-sized iron oxide nanocrystals for high-resolution T1-weighted magnetic resonance imaging. Journal of the American Chemical Society, 133(32), 12624-12631.
- Ferrari, M. (2010). Nanotechnology: what it is, what it isn’t, and why it matters. Nature Reviews Cancer, 10(11), 739-739.
- Rosenblum, D., Joshi, N., Tao, W., Karp, J. M., & Peer, D. (2018). Advancing nanomedicine towards precision medicine. Nature Nanotechnology, 13(3), 190-200.
- Lammers, T., Aime, S., Hennink, W. E., Storm, G., & Kiessling, F. (2011). Theranostic nanomedicine. Accounts of Chemical Research, 44(10), 1057-1068.
- Khan, I., Saeed, K., & Khan, I. (2019). Nanoparticles: Properties, applications and toxicities. Arabian Journal of Chemistry, 12(7), 908-931.
So, future nanoplatforms will be designed by AI? I’m suddenly picturing sentient nanoparticles arguing over the optimal drug delivery route. “Take the lymphatic highway!” “No, the bloodstream is faster, you chrome-plated fool!”
That’s quite the visual! AI-designed nanoplatforms could lead to some interesting optimization debates. Imagine the algorithms battling it out to determine the most efficient and targeted delivery method. The lymphatic highway vs. the bloodstream – it’s the ultimate nano-traffic jam!
Editor: MedTechNews.Uk
Thank you to our Sponsor Esdebe
Considering the potential for personalized medicine through tailored nanoplatforms, how might AI algorithms balance the complex interplay of a patient’s genetics, disease stage, and treatment history to optimize nanoplatform design for truly individualized therapies?
That’s a fantastic question! The integration of AI is key. AI could analyze multi-omic data to predict nanoplatform efficacy. Perhaps AI-driven simulations of nanoplatform interactions within a patient’s unique biological environment could further refine personalized designs. This would allow us to move closer to truly tailored therapies.
Editor: MedTechNews.Uk
Thank you to our Sponsor Esdebe