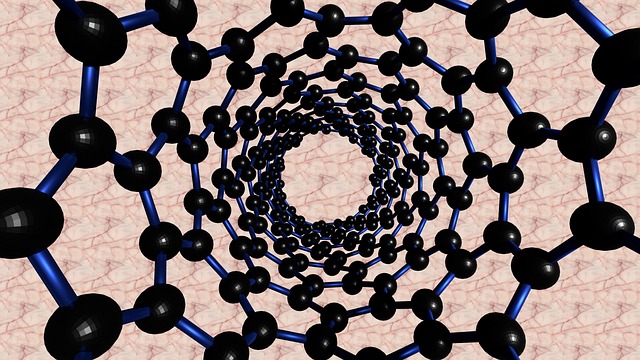
Advanced Sensor Technologies: A Comprehensive Review of Principles, Applications, and Future Directions
Abstract
Sensors are ubiquitous devices that translate physical, chemical, or biological stimuli into measurable signals. Their development and deployment have revolutionized numerous fields, from healthcare and environmental monitoring to industrial automation and consumer electronics. This report presents a comprehensive overview of advanced sensor technologies, exploring their underlying principles, diverse applications, and emerging trends. We delve into various sensor modalities, including physical, chemical, and biosensors, highlighting their operating mechanisms, performance characteristics, and limitations. The report also examines the challenges associated with sensor integration, data processing, and power consumption. Furthermore, we discuss the potential of novel materials, nanotechnology, and artificial intelligence to enhance sensor performance and enable new applications. Finally, we outline the future directions of sensor research, focusing on the development of smart, autonomous, and interconnected sensor networks for a wide range of applications.
1. Introduction
The rapid advancement of microfabrication, materials science, and information technology has fueled the proliferation of sensors across various sectors. Sensors act as the interface between the physical world and electronic systems, providing valuable information for monitoring, control, and decision-making. The ability to accurately and reliably measure a wide range of parameters, such as temperature, pressure, light, sound, chemical composition, and biological activity, has enabled groundbreaking innovations in diverse fields. In healthcare, wearable sensors are transforming patient monitoring and disease management. In environmental science, sensors are deployed to track pollution levels and climate change. In industrial automation, sensors are used to optimize production processes and ensure product quality. And in consumer electronics, sensors are integrated into smartphones, smartwatches, and other devices to provide users with personalized experiences.
The complexity and sophistication of sensor technology have increased dramatically in recent years. Traditional sensors based on simple physical principles have been augmented by advanced sensors that incorporate microelectronics, nanomaterials, and signal processing algorithms. These advanced sensors offer enhanced sensitivity, selectivity, and miniaturization, enabling new applications that were previously impossible. The convergence of sensor technology with other fields, such as artificial intelligence (AI) and the Internet of Things (IoT), has further expanded the potential of sensors. Smart sensors, equipped with embedded processing capabilities, can analyze data locally and make autonomous decisions. Sensor networks, consisting of interconnected sensors, can provide comprehensive monitoring and control of complex systems.
This report aims to provide a comprehensive overview of advanced sensor technologies, encompassing their fundamental principles, diverse applications, and future directions. We will explore the different types of sensors, their operating mechanisms, performance characteristics, and limitations. We will also examine the challenges associated with sensor integration, data processing, and power consumption. Furthermore, we will discuss the potential of novel materials, nanotechnology, and artificial intelligence to enhance sensor performance and enable new applications. Finally, we will outline the future directions of sensor research, focusing on the development of smart, autonomous, and interconnected sensor networks.
2. Sensor Modalities and Principles of Operation
Sensors can be classified based on the physical quantity they measure, the transduction mechanism they employ, or the materials they utilize. This section will delve into the major sensor modalities, discussing their underlying principles of operation, typical performance characteristics, and representative examples.
2.1 Physical Sensors
Physical sensors detect and measure physical properties such as temperature, pressure, force, displacement, acceleration, light, sound, and magnetic fields. These sensors often rely on well-established physical principles and utilize a variety of transduction mechanisms to convert the physical quantity into an electrical signal.
-
Temperature Sensors: Temperature sensors measure the degree of hotness or coldness of an object or environment. Common types include thermocouples, resistance temperature detectors (RTDs), thermistors, and integrated circuit (IC) temperature sensors. Thermocouples generate a voltage proportional to the temperature difference between two dissimilar metals. RTDs exploit the change in electrical resistance of a metal with temperature. Thermistors exhibit a significant change in resistance with temperature, making them suitable for high-sensitivity applications. IC temperature sensors utilize the temperature dependence of semiconductor devices to generate an output voltage or current proportional to temperature.
-
Pressure Sensors: Pressure sensors measure the force exerted by a fluid or gas per unit area. Common types include piezoresistive pressure sensors, capacitive pressure sensors, and resonant pressure sensors. Piezoresistive pressure sensors utilize the change in electrical resistance of a material under mechanical stress. Capacitive pressure sensors measure the change in capacitance between two electrodes due to the deflection of a diaphragm under pressure. Resonant pressure sensors exploit the change in resonant frequency of a vibrating structure with pressure.
-
Accelerometers: Accelerometers measure acceleration, which is the rate of change of velocity. They are widely used in inertial navigation systems, automotive safety systems, and consumer electronics. Common types include piezoelectric accelerometers, piezoresistive accelerometers, and capacitive accelerometers. Piezoelectric accelerometers generate a voltage proportional to the acceleration-induced stress on a piezoelectric crystal. Piezoresistive accelerometers utilize the change in electrical resistance of a material under acceleration-induced stress. Capacitive accelerometers measure the change in capacitance between two electrodes due to the displacement of a proof mass under acceleration.
-
Optical Sensors: Optical sensors detect and measure light. They are used in a wide range of applications, including image sensing, light detection, and optical communication. Common types include photodiodes, phototransistors, and charge-coupled devices (CCDs). Photodiodes generate a current proportional to the incident light intensity. Phototransistors amplify the photodiode current, providing higher sensitivity. CCDs are arrays of light-sensitive elements that convert light into an electrical charge, enabling image capture.
2.2 Chemical Sensors
Chemical sensors detect and measure the presence and concentration of specific chemical species in a gas, liquid, or solid sample. These sensors typically rely on chemical reactions or physical interactions between the analyte and the sensor material. Examples include electrochemical sensors, gas sensors, and optical chemical sensors.
-
Electrochemical Sensors: Electrochemical sensors measure the electrochemical properties of a solution, such as pH, conductivity, and redox potential. Common types include potentiometric sensors, amperometric sensors, and conductometric sensors. Potentiometric sensors measure the potential difference between two electrodes in contact with the solution. Amperometric sensors measure the current flowing through an electrochemical cell at a fixed potential. Conductometric sensors measure the electrical conductivity of the solution.
-
Gas Sensors: Gas sensors detect and measure the concentration of specific gases in the atmosphere. Common types include metal oxide semiconductor (MOS) gas sensors, electrochemical gas sensors, and optical gas sensors. MOS gas sensors utilize the change in electrical conductivity of a metal oxide material upon exposure to a target gas. Electrochemical gas sensors measure the electrochemical oxidation or reduction of a target gas at an electrode. Optical gas sensors measure the absorption or emission of light by a target gas.
2.3 Biosensors
Biosensors are analytical devices that detect and measure the presence and concentration of specific biological molecules, such as DNA, proteins, and cells. They combine a biological recognition element with a transducer that converts the biological signal into a measurable electrical signal. Biosensors are widely used in medical diagnostics, drug discovery, and environmental monitoring. Examples include enzyme-based biosensors, antibody-based biosensors, and DNA-based biosensors.
-
Enzyme-Based Biosensors: Enzyme-based biosensors utilize the catalytic activity of enzymes to detect and measure specific analytes. The enzyme catalyzes a reaction that produces a measurable product, such as a change in pH, oxygen concentration, or electrical current. Glucose biosensors, which use the enzyme glucose oxidase to oxidize glucose, are a well-known example of enzyme-based biosensors.
-
Antibody-Based Biosensors: Antibody-based biosensors utilize the specific binding of antibodies to their target antigens to detect and measure specific biomolecules. The binding of the antibody to the antigen is detected by a transducer, such as a surface plasmon resonance (SPR) sensor or an electrochemical sensor. Antibody-based biosensors are widely used in immunoassays for the detection of diseases and pathogens.
-
DNA-Based Biosensors: DNA-based biosensors utilize the specific hybridization of complementary DNA strands to detect and measure specific DNA sequences. The hybridization of the DNA strands is detected by a transducer, such as a fluorescent sensor or an electrochemical sensor. DNA-based biosensors are used in genetic testing, disease diagnostics, and forensic science.
The choice of sensor modality depends on the specific application, the target analyte, and the required performance characteristics. Each modality has its own advantages and limitations in terms of sensitivity, selectivity, response time, and cost.
3. Key Performance Metrics and Challenges
The performance of a sensor is characterized by several key metrics, including sensitivity, selectivity, accuracy, resolution, response time, and stability. These metrics determine the suitability of a sensor for a specific application. However, achieving optimal performance in all aspects is often challenging due to various factors, such as noise, interference, and environmental conditions.
-
Sensitivity: Sensitivity refers to the change in sensor output per unit change in the input quantity. A high-sensitivity sensor can detect small changes in the input quantity, making it suitable for applications requiring high precision. However, high sensitivity can also make the sensor more susceptible to noise and interference.
-
Selectivity: Selectivity refers to the ability of a sensor to respond specifically to the target analyte in the presence of other interfering substances. A high-selectivity sensor minimizes cross-sensitivity to other analytes, ensuring accurate measurement of the target analyte. Achieving high selectivity can be challenging, especially in complex environments.
-
Accuracy: Accuracy refers to the closeness of the sensor reading to the true value of the input quantity. A high-accuracy sensor provides reliable measurements, which are essential for critical applications. Accuracy can be affected by various factors, such as calibration errors, environmental conditions, and sensor drift.
-
Resolution: Resolution refers to the smallest change in the input quantity that the sensor can detect. A high-resolution sensor can detect very small changes in the input quantity, providing finer-grained measurements. Resolution is often limited by the noise floor of the sensor.
-
Response Time: Response time refers to the time required for the sensor output to reach a specified percentage of its final value after a step change in the input quantity. A fast response time is desirable for applications requiring real-time monitoring or control.
-
Stability: Stability refers to the ability of the sensor to maintain its performance characteristics over time. A stable sensor provides consistent measurements over a long period, reducing the need for frequent recalibration. Stability can be affected by factors such as temperature, humidity, and aging.
Beyond these fundamental performance parameters, other challenges include:
-
Data Quality and Signal Processing: The raw data acquired from sensors can be noisy and may contain artifacts. Signal processing techniques are essential to filter out noise, remove artifacts, and extract meaningful information from the data. Advanced signal processing algorithms, such as Kalman filtering and wavelet analysis, are often used to improve data quality.
-
Power Consumption: Power consumption is a critical concern for battery-powered sensors, such as those used in wearable devices and wireless sensor networks. Minimizing power consumption is essential to extend the battery life of these sensors. Low-power design techniques, such as duty cycling and energy harvesting, are used to reduce power consumption.
-
Miniaturization and Integration: Miniaturization and integration are key trends in sensor technology. Smaller sensors can be easily integrated into portable devices and implantable medical devices. Microfabrication techniques, such as microelectromechanical systems (MEMS) technology, are used to fabricate miniaturized sensors.
-
Cost: Cost is an important consideration for widespread deployment of sensors. Low-cost sensors are essential for applications such as environmental monitoring and consumer electronics. The cost of sensors can be reduced by using inexpensive materials and manufacturing processes.
4. Emerging Trends and Future Directions
The field of sensor technology is constantly evolving, driven by advancements in materials science, nanotechnology, microelectronics, and artificial intelligence. Several emerging trends are shaping the future of sensor technology.
-
Smart Sensors: Smart sensors integrate sensing elements with microprocessors and communication interfaces, enabling them to perform on-board data processing, decision-making, and wireless communication. Smart sensors can adapt to changing environmental conditions, optimize their performance, and communicate with other devices in a network. The development of low-power microprocessors and wireless communication technologies has enabled the widespread adoption of smart sensors.
-
Nanomaterials-Based Sensors: Nanomaterials, such as carbon nanotubes, graphene, and metal nanoparticles, offer unique properties that can enhance sensor performance. Nanomaterials have high surface area, high electrical conductivity, and unique optical properties, making them suitable for developing highly sensitive and selective sensors. Nanomaterials-based sensors are being developed for a wide range of applications, including gas sensing, biosensing, and environmental monitoring.
-
Flexible and Wearable Sensors: Flexible and wearable sensors are designed to conform to the human body or other curved surfaces. These sensors are made from flexible materials, such as polymers and fabrics, and can be integrated into clothing, bandages, or implanted medical devices. Flexible and wearable sensors are being used for a variety of applications, including health monitoring, sports performance analysis, and human-machine interfaces.
-
Wireless Sensor Networks: Wireless sensor networks (WSNs) consist of a large number of interconnected sensors that communicate wirelessly. WSNs can provide comprehensive monitoring and control of complex systems, such as environmental monitoring, industrial automation, and smart cities. WSNs face challenges related to power consumption, data security, and network management.
-
AI-Enabled Sensors: The integration of artificial intelligence (AI) with sensor technology is enabling the development of intelligent sensors that can learn from data, make predictions, and optimize their performance. AI algorithms are used for signal processing, data analysis, and decision-making. AI-enabled sensors are being used in a variety of applications, including predictive maintenance, autonomous vehicles, and personalized healthcare.
-
Energy Harvesting Sensors: The ability to power sensors through energy harvesting, capturing energy from the surrounding environment, offers a solution for self-powered and long-lasting devices. These sensors can utilize sources like solar, thermal, vibration, and radio frequency energy, eliminating the need for frequent battery replacements and enabling continuous monitoring in remote or inaccessible locations.
Looking ahead, the future of sensor technology will be driven by the demand for more accurate, reliable, and cost-effective sensors. The development of novel materials, advanced signal processing algorithms, and AI-enabled sensors will enable new applications and transform various industries. The convergence of sensor technology with other fields, such as IoT, big data analytics, and cloud computing, will further expand the potential of sensors and create new opportunities for innovation.
5. Applications Across Diverse Sectors
The versatility of sensor technology has led to its widespread adoption across numerous sectors. This section highlights some key application areas, showcasing the impact of sensors in various industries.
-
Healthcare: Sensors are revolutionizing healthcare, enabling remote patient monitoring, early disease detection, and personalized treatment. Wearable sensors track vital signs, activity levels, and sleep patterns. Implantable sensors monitor glucose levels, cardiac function, and neurological activity. Biosensors detect biomarkers for cancer, infectious diseases, and other conditions. The convergence of sensor technology with telemedicine and telehealth is transforming healthcare delivery.
-
Environmental Monitoring: Sensors play a crucial role in environmental monitoring, tracking air and water quality, climate change, and natural disasters. Gas sensors detect pollutants in the atmosphere. Water quality sensors measure pH, conductivity, and dissolved oxygen levels. Climate sensors monitor temperature, humidity, and rainfall. Seismic sensors detect earthquakes and volcanic activity. WSNs provide comprehensive environmental monitoring in remote and inaccessible areas.
-
Industrial Automation: Sensors are essential for industrial automation, optimizing production processes, ensuring product quality, and improving worker safety. Temperature sensors monitor the temperature of equipment and processes. Pressure sensors measure the pressure of fluids and gases. Proximity sensors detect the presence of objects. Vision sensors inspect products for defects. Sensors are used in robotics, automated guided vehicles (AGVs), and other automation systems.
-
Agriculture: Sensors are transforming agriculture, enabling precision farming, crop monitoring, and livestock management. Soil moisture sensors measure the moisture content of the soil. Nutrient sensors measure the levels of nutrients in the soil. Weather sensors monitor temperature, humidity, and rainfall. Sensors are used to optimize irrigation, fertilization, and pest control. Animal tracking sensors monitor the location and health of livestock.
-
Aerospace and Defense: Sensors are critical for aerospace and defense applications, providing navigation, surveillance, and threat detection capabilities. Inertial sensors are used in aircraft and spacecraft navigation systems. Radar sensors detect and track targets. Infrared sensors detect heat signatures. Chemical sensors detect explosives and hazardous materials. Sensors are used in drones, missiles, and other defense systems.
-
Automotive: Sensors are integral to modern vehicles, enhancing safety, performance, and fuel efficiency. Accelerometers, gyroscopes, and magnetometers enable advanced driver-assistance systems (ADAS) like electronic stability control and lane departure warning. Oxygen sensors optimize engine combustion, reducing emissions. Pressure sensors monitor tire pressure. Cameras and radar sensors enable adaptive cruise control and automatic emergency braking. With the rise of autonomous vehicles, sensor technology will play an even more critical role in ensuring safe and reliable operation.
6. Conclusion
Sensor technology has undergone remarkable advancements in recent years, driven by innovation in materials science, microfabrication, and information technology. From wearable health monitors to industrial automation systems, sensors are transforming various sectors and improving our daily lives. This report has provided a comprehensive overview of advanced sensor technologies, exploring their underlying principles, diverse applications, and emerging trends. We have discussed the different types of sensors, their operating mechanisms, performance characteristics, and limitations. We have also examined the challenges associated with sensor integration, data processing, and power consumption. Furthermore, we have highlighted the potential of novel materials, nanotechnology, and artificial intelligence to enhance sensor performance and enable new applications.
The future of sensor technology is bright, with ongoing research and development efforts focused on creating smarter, more autonomous, and interconnected sensor networks. These networks will enable a wide range of applications, from personalized healthcare and environmental sustainability to smart cities and autonomous vehicles. As sensor technology continues to evolve, it will play an increasingly important role in shaping our future.
References
[1] D’Orazio, P. (2011). Biosensors in chemical and environmental sensing. John Wiley & Sons.
[2] Fraden, J. (2010). Handbook of modern sensors: physical, design, and application. Springer Science & Business Media.
[3] Madou, M. J., & Tipple, C. A. (2011). Chemical sensors for portable, handheld, and wearable instruments. Chemical Reviews, 111(6), 3144-3221.
[4] Park, S., & Jayaraman, S. (2003). Enhancing the quality of life through wearable technology. IEEE Engineering in Medicine and Biology Magazine, 22(3), 41-48.
[5] Holleman, D., & Bishop, S. (2010). Understanding sensors. Artech House.
[6] Mukhopadhyay, S. C. (2014). Wearable sensors for human activity monitoring: A review. IEEE Sensors Journal, 15(3), 1321-1330.
[7] Marco, S., & Gutierrez-Galvez, A. (2012). Metal oxide semiconductor gas sensors for environmental monitoring. Journal of Sensors, 2012.
[8] Turner, A. P. (2013). Biosensors: sense and sensibility. Chemical Society Reviews, 42(8), 3184-3196.
[9] El-Sheimy, N., Youssef, A., & Lo, S. (2005). Towards ubiquitous positioning: a review of positioning technologies and opportunities. IEEE Potentials, 24(5), 30-35.
[10] Maini, A. K., & Agrawal, V. (2009). Satellite technology: principles and applications. John Wiley & Sons.
[11] Mohammadi, A., Jafari, A., Asadpour, M., & Mobed, A. (2021). Flexible and wearable sensors for healthcare monitoring. Sensors and Actuators A: Physical, 332, 113103.
Given the discussion of AI-enabled sensors, could you elaborate on the ethical considerations surrounding their deployment, particularly regarding data privacy, algorithmic bias, and potential misuse in surveillance applications?
That’s a crucial point! The ethical considerations around AI-enabled sensors, especially concerning data privacy and algorithmic bias, are paramount. As these sensors become more integrated into our lives, we need robust frameworks and regulations to ensure responsible deployment and prevent potential misuse. Let’s discuss the current best practices and how we can improve them!
Editor: MedTechNews.Uk
Thank you to our Sponsor Esdebe