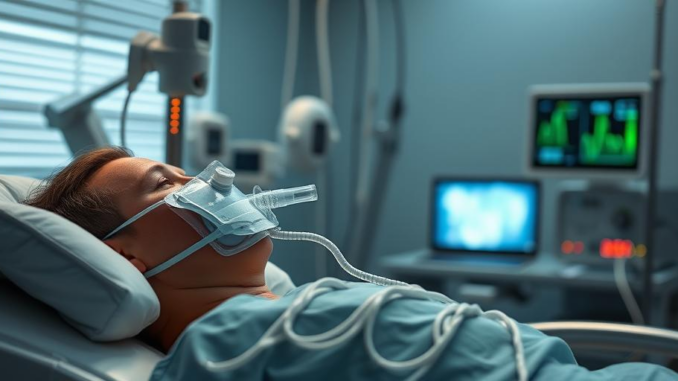
Abstract
Ventilation is a cornerstone of respiratory support for critically ill patients. While mechanical ventilation can be life-saving, it is also associated with a spectrum of complications that can significantly impact patient outcomes. This research report delves into advanced strategies for optimizing ventilation efficacy and minimizing harm in this vulnerable population. We explore the physiological principles underlying various ventilation modes, focusing on personalized approaches tailored to individual patient needs. Furthermore, we examine the intricate relationship between ventilator settings, lung mechanics, and gas exchange, emphasizing the importance of continuous monitoring and data-driven adjustments. Emerging technologies, such as closed-loop ventilation systems and artificial intelligence-powered decision support tools, are discussed in the context of improving ventilation management. Finally, we address strategies for preventing ventilator-associated complications, including ventilator-associated pneumonia (VAP) and ventilator-induced lung injury (VILI), incorporating the latest evidence-based guidelines and innovative preventative measures. The report concludes with a discussion of future directions in ventilation research and clinical practice, highlighting the potential for personalized and adaptive ventilation strategies to revolutionize respiratory support in critically ill patients.
Many thanks to our sponsor Esdebe who helped us prepare this research report.
1. Introduction
Mechanical ventilation serves as a critical intervention for patients experiencing respiratory failure or severe respiratory distress. While it is often life-saving, the use of mechanical ventilation can lead to various complications that can potentially negate its benefits and prolong the patient’s stay in the intensive care unit (ICU). This report aims to provide an in-depth exploration of the advanced strategies employed to improve ventilation efficacy and minimize associated complications in critically ill patients. We delve into the physiological principles underpinning different ventilation modes, emphasizing the importance of tailoring ventilation strategies to individual patient needs based on their underlying pathophysiology and lung mechanics. Furthermore, we examine the intricate relationship between ventilator settings, lung mechanics, and gas exchange, emphasizing the role of continuous monitoring and data-driven adjustments in optimizing ventilation. Finally, we explore the latest evidence-based guidelines and innovative preventative measures for reducing ventilator-associated complications, such as VAP and VILI. This report aims to provide a comprehensive understanding of the current state of the art in mechanical ventilation and highlight potential future directions for enhancing respiratory support in critically ill patients.
Many thanks to our sponsor Esdebe who helped us prepare this research report.
2. Principles of Mechanical Ventilation: A Physiological Perspective
Mechanical ventilation works by delivering breaths to the patient, assisting or replacing their own respiratory effort. Understanding the fundamental physiological principles governing gas exchange and lung mechanics is crucial for optimizing ventilator settings and minimizing lung injury. Minute ventilation (VE), the product of tidal volume (VT) and respiratory rate (RR), dictates carbon dioxide (CO2) elimination. However, increasing VE excessively can lead to overdistention and alveolar damage.
2.1. Lung Mechanics and Compliance
Lung compliance, a measure of the lung’s ability to expand under pressure, is a key determinant of ventilator settings. In patients with acute respiratory distress syndrome (ARDS), lung compliance is often reduced due to alveolar edema, inflammation, and atelectasis. Ventilating these patients with high tidal volumes can lead to VILI. Protective ventilation strategies, employing lower tidal volumes (6-8 mL/kg predicted body weight) and higher positive end-expiratory pressure (PEEP), have been shown to improve outcomes in ARDS [1].
2.2. Gas Exchange and Dead Space
The efficiency of gas exchange is determined by the matching of ventilation and perfusion (V/Q ratio) in the lungs. Dead space refers to the volume of inspired air that does not participate in gas exchange. Anatomical dead space is the volume of the conducting airways, while alveolar dead space is the volume of alveoli that are ventilated but not perfused. Increasing dead space reduces the efficiency of gas exchange, requiring a higher VE to maintain adequate PaCO2. In patients with pulmonary embolism or severe lung disease, dead space may be significantly increased, requiring careful titration of ventilator settings to optimize gas exchange.
2.3. Pressure-Volume Loops and Respiratory Mechanics Monitoring
Pressure-volume (PV) loops provide a graphical representation of lung mechanics during mechanical ventilation. Analyzing the shape of the PV loop can provide valuable insights into lung compliance, resistance, and the presence of overdistention or alveolar collapse. For example, a “beak” in the PV loop suggests overdistention, while a flattening of the loop indicates alveolar collapse. Advanced respiratory mechanics monitoring, including measurements of plateau pressure, driving pressure, and dynamic compliance, can help clinicians optimize ventilator settings and prevent VILI [2]. Driving pressure, defined as the difference between plateau pressure and PEEP, has emerged as a key predictor of mortality in ARDS patients [3].
Many thanks to our sponsor Esdebe who helped us prepare this research report.
3. Ventilation Modes: A Spectrum of Options
Various ventilation modes are available, each with its own advantages and disadvantages. Selecting the appropriate mode depends on the patient’s respiratory drive, lung mechanics, and overall clinical condition.
3.1. Volume Control Ventilation (VCV)
In VCV, the ventilator delivers a preset tidal volume with each breath. This mode ensures a consistent tidal volume, regardless of changes in lung compliance or resistance. However, VCV can lead to high airway pressures, especially in patients with reduced lung compliance. Pressure monitoring is crucial to avoid overdistention and VILI.
3.2. Pressure Control Ventilation (PCV)
In PCV, the ventilator delivers a breath at a preset pressure. The tidal volume delivered varies depending on the patient’s lung compliance and resistance. PCV can improve patient comfort and reduce the risk of overdistention compared to VCV. However, the delivered tidal volume may be inconsistent, requiring careful monitoring of VE.
3.3. Pressure Support Ventilation (PSV)
PSV is a spontaneous breathing mode in which the ventilator provides pressure support to augment the patient’s inspiratory effort. PSV can reduce the work of breathing and improve patient comfort. It is commonly used during weaning from mechanical ventilation. The level of pressure support is titrated to achieve a target tidal volume and respiratory rate.
3.4. Synchronized Intermittent Mandatory Ventilation (SIMV)
SIMV combines mandatory breaths with spontaneous breaths. The ventilator delivers a preset number of mandatory breaths, while the patient can breathe spontaneously between the mandatory breaths. SIMV can be used in conjunction with PSV to provide additional support during spontaneous breaths. This mode is often used as a transitional mode during weaning.
3.5. Airway Pressure Release Ventilation (APRV)
APRV is a pressure-controlled mode that allows spontaneous breathing at two levels of continuous positive airway pressure (CPAP). The higher pressure (P high) is maintained for a longer duration (T high), while the lower pressure (P low) is maintained for a shorter duration (T low). APRV can improve oxygenation and reduce VILI by promoting alveolar recruitment and reducing cyclic alveolar collapse. APRV can be challenging to manage and requires careful monitoring of respiratory mechanics.
3.6. Neurally Adjusted Ventilatory Assist (NAVA)
NAVA is a ventilation mode that delivers pressure support in proportion to the patient’s neural respiratory drive. The ventilator detects the electrical activity of the diaphragm (Edi) and delivers pressure support in response. NAVA can improve patient-ventilator synchrony and reduce the risk of over-assistance or under-assistance. NAVA requires the placement of a specialized Edi catheter.
Many thanks to our sponsor Esdebe who helped us prepare this research report.
4. Complications of Mechanical Ventilation: Prevention and Management
Mechanical ventilation is associated with a range of complications that can adversely affect patient outcomes. Prevention is crucial for minimizing the risk of these complications.
4.1. Ventilator-Associated Pneumonia (VAP)
VAP is a common and serious complication of mechanical ventilation. It is defined as pneumonia that develops more than 48 hours after intubation. VAP is associated with increased morbidity, mortality, and healthcare costs. Strategies for preventing VAP include: maintaining proper hand hygiene; elevating the head of the bed to 30-45 degrees; using continuous subglottic secretion drainage; minimizing sedation; and promoting early mobilization [4]. Selective digestive decontamination (SDD) and selective oropharyngeal decontamination (SOD) have also been shown to reduce the risk of VAP in some patient populations [5]. However, the use of SDD and SOD remains controversial due to concerns about antibiotic resistance.
4.2. Ventilator-Induced Lung Injury (VILI)
VILI encompasses a spectrum of lung injuries caused by mechanical ventilation. These injuries include: barotrauma (lung injury due to excessive airway pressure); volutrauma (lung injury due to excessive tidal volume); atelectotrauma (lung injury due to repeated alveolar collapse and re-expansion); and biotrauma (lung injury due to the release of inflammatory mediators). Protective ventilation strategies, employing lower tidal volumes (6-8 mL/kg predicted body weight) and higher PEEP, have been shown to reduce the risk of VILI. Monitoring driving pressure and plateau pressure is crucial for preventing overdistention and alveolar damage. In patients with severe ARDS, prone positioning can improve oxygenation and reduce mortality by redistributing lung perfusion and improving ventilation-perfusion matching [6].
4.3. Diaphragm Dysfunction
Prolonged mechanical ventilation can lead to diaphragm dysfunction, characterized by muscle atrophy and weakness. Diaphragm dysfunction can prolong weaning from mechanical ventilation and increase the risk of respiratory failure after extubation. Strategies for preventing diaphragm dysfunction include: minimizing sedation; using spontaneous breathing modes; and implementing early mobilization and rehabilitation [7]. Phrenic nerve stimulation has also shown promise in preventing diaphragm atrophy during mechanical ventilation, although more research is needed to confirm its efficacy [8].
4.4. Cardiovascular Complications
Mechanical ventilation can affect cardiovascular function by increasing intrathoracic pressure and reducing venous return. High intrathoracic pressure can decrease cardiac output and blood pressure. PEEP can also impair cardiac function by reducing venous return. Careful monitoring of hemodynamic parameters, such as blood pressure, heart rate, and cardiac output, is crucial during mechanical ventilation. Fluid management is also important for maintaining adequate preload and preventing pulmonary edema.
4.5. Other Complications
Other complications of mechanical ventilation include: tracheal stenosis; tracheoesophageal fistula; sinusitis; and delirium. Regular monitoring and preventative measures are essential for minimizing the risk of these complications. Delirium, in particular, is a common and serious complication of mechanical ventilation. Strategies for preventing delirium include: minimizing sedation; promoting sleep; and implementing early mobilization and cognitive stimulation [9].
Many thanks to our sponsor Esdebe who helped us prepare this research report.
5. Optimizing Ventilation Settings: A Personalized Approach
Optimizing ventilation settings requires a personalized approach tailored to the individual patient’s needs. This involves considering the patient’s underlying pathophysiology, lung mechanics, and overall clinical condition. Continuous monitoring of respiratory mechanics, gas exchange, and hemodynamic parameters is essential for making informed decisions about ventilator settings.
5.1. Titrating Tidal Volume and PEEP
Tidal volume and PEEP are two of the most important ventilator settings. The goal is to deliver a tidal volume that provides adequate ventilation without causing overdistention or alveolar damage. PEEP is used to prevent alveolar collapse and improve oxygenation. The optimal level of PEEP varies depending on the patient’s lung mechanics and oxygenation status. Several methods have been proposed for titrating PEEP, including: using the lowest PEEP that provides adequate oxygenation; using the PEEP that results in the best lung compliance; and using the PEEP that minimizes driving pressure [10].
5.2. Managing Airway Pressure
Airway pressure should be carefully monitored to avoid barotrauma and volutrauma. Plateau pressure, a measure of alveolar pressure at the end of inspiration, should be kept below 30 cm H2O. Driving pressure, the difference between plateau pressure and PEEP, should be minimized to reduce the risk of VILI. If airway pressures are high, strategies such as reducing tidal volume, increasing PEEP, or changing ventilation mode may be necessary.
5.3. Optimizing Oxygenation and Ventilation
Oxygenation and ventilation should be optimized to maintain adequate arterial blood gas values. The target PaO2 and PaCO2 values vary depending on the patient’s underlying condition. In general, a PaO2 of 60-80 mmHg and a PaCO2 of 35-45 mmHg are considered acceptable. However, in patients with chronic obstructive pulmonary disease (COPD), a higher PaCO2 may be tolerated. The FiO2 should be adjusted to maintain adequate oxygenation while minimizing the risk of oxygen toxicity.
5.4. Closed-Loop Ventilation Systems
Closed-loop ventilation systems automatically adjust ventilator settings based on patient feedback. These systems can improve patient-ventilator synchrony and reduce the work of breathing. Examples of closed-loop ventilation systems include: automatic tube compensation (ATC); adaptive support ventilation (ASV); and proportional assist ventilation (PAV). Closed-loop ventilation systems have been shown to reduce the duration of mechanical ventilation in some patient populations [11].
5.5. Artificial Intelligence-Powered Decision Support
Artificial intelligence (AI) is increasingly being used to develop decision support tools for mechanical ventilation. These tools can analyze patient data and provide recommendations for ventilator settings. AI-powered decision support tools have the potential to improve ventilation management and reduce the risk of complications. However, more research is needed to validate their efficacy and safety [12].
Many thanks to our sponsor Esdebe who helped us prepare this research report.
6. Future Directions in Ventilation Research and Clinical Practice
The field of mechanical ventilation is constantly evolving. Future research will likely focus on developing more personalized and adaptive ventilation strategies, as well as on improving the prevention and management of ventilator-associated complications. Some potential future directions include:
- Personalized Ventilation Strategies: Tailoring ventilation settings to individual patient characteristics and lung mechanics.
- Adaptive Ventilation Modes: Developing ventilation modes that automatically adjust to changes in patient condition.
- Advanced Monitoring Techniques: Using advanced monitoring techniques, such as electrical impedance tomography (EIT) and lung ultrasound, to guide ventilator settings.
- Pharmacological Interventions: Developing pharmacological interventions to reduce inflammation and promote lung healing.
- Non-Invasive Ventilation: Expanding the use of non-invasive ventilation to avoid intubation and reduce the risk of VAP.
- Extracorporeal Membrane Oxygenation (ECMO): Using ECMO as a rescue therapy for patients with severe respiratory failure.
- Artificial Intelligence and Machine Learning: Utilizing AI and machine learning to optimize ventilation management and predict patient outcomes.
Many thanks to our sponsor Esdebe who helped us prepare this research report.
7. Conclusion
Mechanical ventilation is a complex and challenging intervention that requires careful consideration of the patient’s individual needs. By understanding the physiological principles underlying ventilation, employing appropriate ventilation modes, preventing ventilator-associated complications, and optimizing ventilation settings, clinicians can improve patient outcomes and reduce the burden of respiratory failure. Future research will continue to refine ventilation strategies and develop new technologies to enhance respiratory support in critically ill patients.
Many thanks to our sponsor Esdebe who helped us prepare this research report.
References
[1] ARDSNet. (2000). Ventilation with lower tidal volumes as compared with traditional tidal volumes for acute lung injury and the acute respiratory distress syndrome. The New England Journal of Medicine, 342(18), 1301-1308.
[2] Gattinoni, L., Quintel, M., & Roupie, E. (2005). Mechanical ventilation viewed from the ventilator. Critical Care Medicine, 33(5), 1147-1154.
[3] Amato, M. B. P., Meade, M. O., Slutsky, A. S., Brochard, L., Costa, E. L. V., Schoenfeld, D. A., … & PROVE Network Investigators. (2015). Driving pressure and survival in the acute respiratory distress syndrome. The New England Journal of Medicine, 372(8), 747-755.
[4] Klompas, M., Branson, R., Eichenwald, E. C., Greene, L. R., Howell, M. D., Kaplan, L. J., … & Yokoe, D. S. (2014). Strategies to prevent ventilator-associated pneumonia in acute care hospitals: 2014 update. Infection Control & Hospital Epidemiology, 35(S2), S133-S154.
[5] Silvestri, L., van Saene, H. K. F., Petrini, F., & Monti, P. (2013). Selective digestive decontamination in intensive care unit patients: a systematic review of randomized controlled trials. Journal of Chemotherapy, 25(2), 65-74.
[6] Guérin, C., Reignier, J., Richard, J. C., Beuret, P., Gacouin, A., Boulain, T., … & PROSEVA Study Group. (2013). Prone positioning in severe acute respiratory distress syndrome. The New England Journal of Medicine, 368(23), 2159-2168.
[7] Levine, S., Nguyen, T., Taylor, N., Friscia, M. E., Gamelli, R. L., Grap, M. J., … & Marcucci, M. (2008). Rapid disuse atrophy of diaphragm fibers in mechanically ventilated humans. The New England Journal of Medicine, 358(13), 1327-1335.
[8] Gayan-Ramirez, G., Testelmans, D., Maes, K., Verleden, G., Ruttens, D., Schols, D., … & Decramer, M. (2009). Intermittent phrenic nerve stimulation prevents diaphragm atrophy during mechanical ventilation in dogs. Critical Care Medicine, 37(9), 2593-2601.
[9] Ely, E. W., Shintani, A., Truman, B., Speroff, T., Gordon, S. M., Harrell, F. E., Jr, … & Dittus, R. S. (2004). Delirium as a predictor of mortality in mechanically ventilated patients in the intensive care unit. JAMA, 291(14), 1753-1762.
[10] Mercat, A., Richard, J. C. M., Vielle, B., Jaber, S., Osman, D., Diehl, J. L., … & Exolung Study Group. (2008). Positive end-expiratory pressure setting in adults with acute lung injury and acute respiratory distress syndrome: a randomized controlled trial. JAMA, 299(6), 646-655.
[11] Brochard, L., Lellouche, F., Hill, N. S., Maggiore, S. M., Mercat, A., Richard, J. C., … & Mancebo, J. (2013). Automated closed-loop ventilation: state of the art. Critical Care, 17(3), 314.
[12] Moor, M., Persson, M., & Barach, P. (2021). The role of artificial intelligence in mechanical ventilation. Critical Care, 25(1), 1-11.
Protective ventilation strategies, huh? Lower tidal volumes, higher PEEP… sounds like a recipe for a calmer ICU. Wonder if we could apply similar “less is more” philosophies to other areas of critical care? Maybe fewer alarms? Just a thought!
That’s a great point! Thinking about “less is more” regarding alarms could be really beneficial. Perhaps refining alarm settings to focus on clinically significant events, rather than every minor deviation, could reduce alarm fatigue and improve clinician response times. It’s definitely worth exploring! What are your thoughts about what might be clinically significant?
Editor: MedTechNews.Uk
Thank you to our Sponsor Esdebe