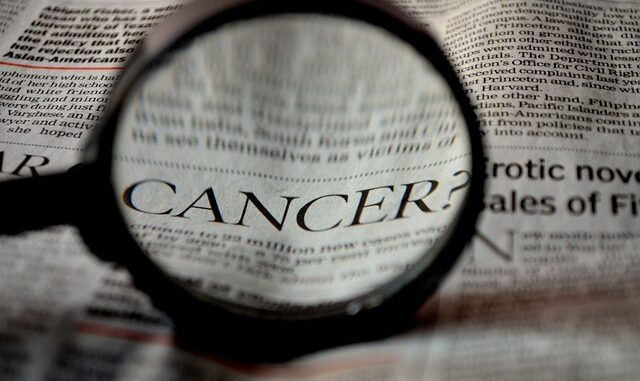
Advancements and Future Directions in Radiation Therapy: A Comprehensive Overview
Abstract
Radiation therapy, a cornerstone of cancer treatment, continues to evolve rapidly, driven by technological innovations and a deeper understanding of tumor biology. This report provides a comprehensive overview of recent advancements in radiation therapy, encompassing improvements in treatment delivery, enhanced imaging modalities for precise targeting, and strategies for mitigating treatment-related toxicities. We delve into specific techniques such as intensity-modulated radiation therapy (IMRT), stereotactic body radiation therapy (SBRT), proton therapy, and brachytherapy, examining their applications across various cancer types. Furthermore, we explore emerging trends, including the integration of artificial intelligence (AI) and machine learning (ML) for personalized treatment planning, the development of novel radiosensitizers, and the exploration of FLASH radiotherapy. Finally, we discuss the challenges and future directions in the field, highlighting the need for continued research and collaboration to optimize treatment outcomes and improve the quality of life for cancer patients.
1. Introduction
Radiation therapy utilizes ionizing radiation to damage the DNA of cancer cells, leading to their death or impaired ability to proliferate. While its fundamental principle remains the same, the field of radiation therapy has undergone significant transformations in recent decades. These advancements are fueled by several factors: improved understanding of radiobiology, advances in imaging technologies, sophisticated computer algorithms for treatment planning, and innovative delivery systems. These combined advances have allowed for more precise radiation targeting, sparing healthy tissues and delivering higher doses to tumors, thereby improving local control rates and reducing side effects. Given Elekta’s role in developing and implementing these technologies, it is essential to understand the broader landscape of radiation therapy advancements.
Traditional radiation therapy involved broad beams of radiation delivered from external sources, often leading to significant collateral damage to surrounding healthy tissues. Modern techniques, such as IMRT and SBRT, have revolutionized the field by allowing radiation oncologists to shape the radiation beam precisely to conform to the tumor volume while minimizing exposure to critical organs. Furthermore, advances in imaging, including computed tomography (CT), magnetic resonance imaging (MRI), and positron emission tomography (PET), have enabled more accurate tumor delineation and treatment planning.
This report aims to provide a comprehensive overview of the current state of radiation therapy, focusing on the latest advancements, emerging trends, and future directions. We will discuss the various modalities of radiation therapy, their applications in different cancer types, and the challenges associated with their implementation. We will also explore the role of technology in advancing the field, with a particular emphasis on the integration of AI and ML.
2. External Beam Radiation Therapy (EBRT) Techniques
EBRT is the most common form of radiation therapy, where radiation is delivered from a source external to the body. Several advanced EBRT techniques have emerged, each with its own advantages and limitations.
2.1 Intensity-Modulated Radiation Therapy (IMRT)
IMRT is a sophisticated technique that allows for the delivery of non-uniform radiation doses to the tumor, enabling better sparing of surrounding healthy tissues. IMRT utilizes computer-controlled linear accelerators to modulate the intensity of the radiation beam, creating complex dose distributions that conform precisely to the tumor volume. This is achieved by dividing the radiation beam into multiple smaller beamlets, each with its own intensity. The intensity of each beamlet is optimized using sophisticated algorithms to achieve the desired dose distribution.
The main advantage of IMRT is its ability to deliver higher doses to the tumor while minimizing exposure to critical organs. This has led to improved local control rates and reduced side effects in various cancer types, including prostate cancer, head and neck cancer, and breast cancer [1]. However, IMRT is more complex and time-consuming than conventional radiation therapy, requiring specialized equipment and expertise. Furthermore, IMRT may result in a slightly higher integral dose to the patient, potentially increasing the risk of secondary cancers in the long term.
2.2 Volumetric Modulated Arc Therapy (VMAT)
VMAT is an advanced form of IMRT that delivers radiation while the linear accelerator rotates continuously around the patient. This allows for faster treatment delivery compared to IMRT, reducing the overall treatment time. VMAT also offers the potential for improved dose conformity and target coverage [2].
The main advantage of VMAT is its efficiency. The faster treatment delivery can improve patient comfort and reduce the risk of motion artifacts. However, VMAT requires precise synchronization of the gantry rotation, beam intensity modulation, and multi-leaf collimator (MLC) movement, which can be technically challenging. Like IMRT, VMAT also carries a risk of increased integral dose compared to conventional techniques.
2.3 Stereotactic Radiosurgery (SRS) and Stereotactic Body Radiation Therapy (SBRT)
SRS and SBRT are highly precise radiation therapy techniques that deliver high doses of radiation in a small number of fractions (typically 1-5 fractions) to well-defined targets. SRS is typically used to treat intracranial lesions, while SBRT is used to treat extracranial tumors in various organs, including the lung, liver, and prostate. These techniques rely on precise image guidance and immobilization to ensure accurate targeting and minimize exposure to surrounding healthy tissues [3].
The main advantage of SRS and SBRT is their ability to deliver ablative doses of radiation to tumors in a short period of time. This can lead to high local control rates and improved survival in selected patients. However, SRS and SBRT require specialized equipment and expertise and are associated with a higher risk of complications if not performed accurately. Patient selection is critical, and careful consideration should be given to the tumor size, location, and proximity to critical organs.
2.4 Proton Therapy
Proton therapy is a form of particle therapy that uses protons instead of photons (X-rays) to deliver radiation. Protons have a unique property called the Bragg peak, where they deposit most of their energy at a specific depth in the tissue, with minimal dose beyond that point. This allows for more precise targeting of the tumor and reduced exposure to surrounding healthy tissues [4].
The main advantage of proton therapy is its potential to reduce radiation-related side effects, particularly in children and patients with tumors located near critical organs. However, proton therapy is more expensive and less widely available than conventional photon therapy. Furthermore, the range of protons in tissue can be affected by variations in tissue density, which can lead to uncertainties in dose delivery. Advancements are continually being made in areas such as pencil beam scanning proton therapy, which allows for even greater conformity of the proton dose to the tumor.
3. Brachytherapy
Brachytherapy involves placing radioactive sources directly into or near the tumor. This allows for the delivery of high doses of radiation to the tumor while sparing surrounding healthy tissues. Brachytherapy can be delivered in various forms, including intracavitary, interstitial, and surface brachytherapy [5].
3.1 High-Dose-Rate (HDR) Brachytherapy
HDR brachytherapy involves the temporary placement of a high-activity radioactive source near the tumor. The source is then removed after a short period of time, minimizing the risk of radiation exposure to healthcare personnel. HDR brachytherapy is commonly used to treat gynecological cancers, prostate cancer, and breast cancer.
The main advantage of HDR brachytherapy is its ability to deliver high doses of radiation in a short period of time. This can lead to improved local control rates and reduced treatment time. However, HDR brachytherapy requires specialized equipment and expertise and is associated with a risk of complications such as bleeding, infection, and damage to surrounding tissues.
3.2 Low-Dose-Rate (LDR) Brachytherapy
LDR brachytherapy involves the permanent placement of low-activity radioactive seeds into the tumor. The seeds gradually release radiation over a period of weeks or months, providing continuous radiation exposure to the tumor. LDR brachytherapy is commonly used to treat prostate cancer.
The main advantage of LDR brachytherapy is its convenience and ease of administration. However, LDR brachytherapy is associated with a risk of seed migration and potential radiation exposure to family members and caregivers. Stringent radiation safety protocols are necessary.
4. Imaging in Radiation Therapy
Imaging plays a crucial role in all stages of radiation therapy, from diagnosis and staging to treatment planning and response assessment. Accurate tumor delineation and precise targeting are essential for maximizing treatment efficacy and minimizing side effects. Several imaging modalities are used in radiation therapy, each with its own strengths and limitations.
4.1 Computed Tomography (CT)
CT is the most commonly used imaging modality in radiation therapy. CT provides high-resolution anatomical images that are used for tumor delineation and treatment planning. CT scans are typically acquired with intravenous contrast to enhance the visualization of tumors and surrounding structures.
4.2 Magnetic Resonance Imaging (MRI)
MRI provides superior soft tissue contrast compared to CT, making it particularly useful for delineating tumors in the brain, prostate, and other soft tissues. MRI can also provide functional information, such as perfusion and diffusion, which can be used to assess tumor response to treatment.
4.3 Positron Emission Tomography (PET)
PET uses radioactive tracers to detect metabolic activity in the body. PET scans are commonly used to stage cancers and assess tumor response to treatment. PET/CT combines PET and CT imaging to provide both anatomical and functional information. In particular, the PET tracers FDG (Fluorodeoxyglucose) can be used to measure the metabolism within a cell. More recently, other tracers are becoming more available such as PSMA which is more specific to prostate cancer.
4.4 Image-Guided Radiation Therapy (IGRT)
IGRT refers to the use of imaging during radiation therapy to verify the position of the tumor and ensure accurate targeting. IGRT can be performed using various imaging modalities, including CT, cone-beam CT (CBCT), and ultrasound. IGRT allows for real-time adjustments to the treatment plan to compensate for patient movement and anatomical changes, improving treatment accuracy [6].
5. Management of Side Effects
Radiation therapy can cause various side effects, depending on the location and dose of radiation. These side effects can range from mild to severe and can significantly impact the patient’s quality of life. Effective management of side effects is essential for optimizing treatment outcomes and improving patient satisfaction.
5.1 Acute Side Effects
Acute side effects occur during or shortly after radiation therapy. Common acute side effects include skin reactions, fatigue, nausea, vomiting, and mucositis. These side effects are typically temporary and resolve within a few weeks or months after treatment completion. Symptomatic treatment is often used to manage acute side effects. Topical creams and emollients can be used to soothe skin reactions. Anti-emetics can be used to prevent nausea and vomiting. Pain medications can be used to manage mucositis.
5.2 Late Side Effects
Late side effects occur months or years after radiation therapy. Common late side effects include fibrosis, lymphedema, and secondary cancers. Late side effects are often irreversible and can have a significant impact on the patient’s long-term quality of life. Prevention is key to minimizing the risk of late side effects. This can be achieved by using advanced radiation therapy techniques to minimize exposure to healthy tissues. Early detection and management of late side effects can also help to improve outcomes.
5.3 Supportive Care
Supportive care plays a crucial role in managing side effects and improving the patient’s overall well-being. Supportive care includes nutritional counseling, pain management, physical therapy, and psychosocial support. A multidisciplinary approach involving radiation oncologists, nurses, therapists, and social workers is essential for providing comprehensive supportive care.
6. Emerging Trends and Future Directions
The field of radiation therapy is constantly evolving, with new technologies and techniques being developed to improve treatment outcomes and reduce side effects. Several emerging trends are poised to shape the future of radiation therapy.
6.1 Artificial Intelligence (AI) and Machine Learning (ML)
AI and ML are increasingly being used in radiation therapy for various applications, including treatment planning, image segmentation, and response prediction. AI algorithms can automate the process of treatment planning, reducing the time and effort required to create optimal treatment plans. ML models can be trained to predict tumor response to radiation therapy, allowing for personalized treatment strategies. Furthermore, AI can aid in automated image segmentation, reducing the inter-observer variability and improving the accuracy of tumor delineation [7].
6.2 FLASH Radiotherapy
FLASH radiotherapy is an emerging technique that delivers radiation at ultra-high dose rates (greater than 40 Gy/s). Preclinical studies have shown that FLASH radiotherapy can spare normal tissues while maintaining tumor control. The mechanisms underlying the FLASH effect are not fully understood, but it is believed to involve the depletion of oxygen in normal tissues. Clinical trials are currently underway to evaluate the safety and efficacy of FLASH radiotherapy in humans [8].
6.3 Radiosensitizers
Radiosensitizers are drugs that enhance the effects of radiation on cancer cells. Several radiosensitizers are currently being investigated in clinical trials, including chemotherapeutic agents, targeted therapies, and immune checkpoint inhibitors. The combination of radiation therapy and radiosensitizers has the potential to improve local control rates and survival in various cancer types [9].
6.4 Adaptive Radiotherapy
Adaptive radiotherapy involves modifying the treatment plan during the course of radiation therapy based on changes in tumor size, shape, or location. This allows for more precise targeting of the tumor and reduced exposure to surrounding healthy tissues. Adaptive radiotherapy requires frequent imaging and sophisticated treatment planning software.
6.5 Personalized Radiation Therapy
Personalized radiation therapy involves tailoring the treatment plan to the individual patient based on their unique tumor biology, genetic profile, and clinical characteristics. This requires the integration of data from various sources, including imaging, genomics, and proteomics. Personalized radiation therapy has the potential to improve treatment outcomes and reduce side effects [10].
7. Conclusion
Radiation therapy continues to be a vital component of cancer care, with ongoing advancements significantly improving treatment efficacy and reducing side effects. Techniques such as IMRT, SBRT, and proton therapy have revolutionized the field, enabling more precise targeting and higher doses to tumors. The integration of advanced imaging modalities, AI, and ML is further enhancing treatment planning and personalization. Emerging trends like FLASH radiotherapy and the development of novel radiosensitizers hold great promise for future improvements. However, challenges remain in terms of accessibility, cost, and the need for continued research to optimize treatment protocols and manage long-term side effects. By focusing on these areas, the field of radiation therapy can continue to evolve and provide even better outcomes for cancer patients.
References
[1] Nutting, C. M., Morden, J. P., Harrington, K. J., Urbano, T. G., Bhide, S. A., … & Moore, C. J. (2011). Parotid-sparing intensity modulated versus conventional radiotherapy in head and neck cancer (PARSPORT): a phase 3 multicentre randomised controlled trial. The Lancet Oncology, 12(2), 127-136.
[2] Van Gestel, D., Van den Begin, R., Michielsen, D., De Ost, B., Van Damme, N., Goor, C., … & Weltens, C. (2018). Volumetric modulated arc therapy versus intensity-modulated radiotherapy for head and neck cancer: A systematic review and meta-analysis. International Journal of Radiation Oncology• Biology• Physics, 101(2), 307-318.
[3] Timmerman, R., Kavanagh, B., Cho, L. C., Dawson, L., Li, X., Flickinger, J., … & Fakiris, A. J. (2011). Stereotactic body radiation therapy in multiple organ sites. Journal of Clinical Oncology, 28(32), 4854-4862.
[4] Paganetti, H. (2012). Proton therapy physics. Medical Physics, 39(8), 4708-4737.
[5] Nag, S., Cardenes, H. R., Chang, S., Varia, M., & Fowler, J. F. (2003). American Brachytherapy Society recommendations for high-dose-rate brachytherapy for carcinoma of the cervix. International Journal of Radiation Oncology• Biology• Physics, 57(3), 633-643.
[6] Jaffray, D. A. (2012). Image-guided radiotherapy: from current technology to future directions. The Lancet Oncology, 13(9), e461-e469.
[7] Sibindi, T. A., Pretorius, P. H., & Madala, N. E. (2021). A review of artificial intelligence in radiation therapy: current status and future directions. Artificial Intelligence in Medicine, 117, 102097.
[8] Favaudon, V., Fouquet, G., Méry, B., Vozenin, M. C., Gegner, E., Patin, D., … & Bourhis, J. (2014). Ultrahigh dose rate FLASH irradiation increases the differential response between normal and tumor tissue in mice. Science Translational Medicine, 6(259), 259ra147.
[9] Baumann, M., Krause, M., Hill, R., Dubben, H. H., Dörr, W., & Friedmann, J. (2016). Hypoxia and radiation resistance: novel strategies for cancer therapy. Strahlentherapie und Onkologie, 192(11), 831-839.
[10] West, C. M. L., & Rosenstein, B. S. (2010). Individualizing cancer treatment: the role of predictive biomarkers. Seminars in Radiation Oncology, 20(2), 83-90.
The integration of AI and ML for personalized treatment planning is intriguing. How might these technologies address the challenges of inter-patient tumor heterogeneity and varying responses to radiation, ultimately leading to more tailored and effective cancer therapies?
That’s a great point! AI and ML could analyze vast datasets of patient-specific tumor characteristics (genomics, imaging, etc.) to predict individual responses. This could allow us to customize radiation doses and techniques, potentially overcoming heterogeneity and optimizing outcomes for each patient. This type of collaborative approach is key!
Editor: MedTechNews.Uk
Thank you to our Sponsor Esdebe
Given the increasing sophistication of imaging techniques like PET/CT, how might we better integrate real-time metabolic data into adaptive radiotherapy plans to personalize treatment and account for tumor heterogeneity during treatment?
That’s an excellent question! The potential for PET/CT to inform adaptive radiotherapy is huge. Imagine using real-time metabolic data to dynamically adjust radiation fields, focusing on the most active tumor regions while sparing healthy tissue. This could significantly improve treatment efficacy and reduce toxicity. What are your thoughts on the logistical challenges of implementing this?
Editor: MedTechNews.Uk
Thank you to our Sponsor Esdebe
Fascinating overview! Though with all the talk of high-tech solutions, does anyone consider the simple things? Like ensuring equitable access to these advancements, regardless of socioeconomic status or geographic location? Or are we just building fancier toys for the privileged few?
Thanks for raising this important point. Equitable access is vital! It’s not just about fancy tech, but also about how we implement and distribute it. Perhaps more collaborative initiatives between research institutions, governments, and community organizations could help bridge the gap and ensure these advancements benefit all patients, regardless of their background or location. How can we make this happen?
Editor: MedTechNews.Uk
Thank you to our Sponsor Esdebe