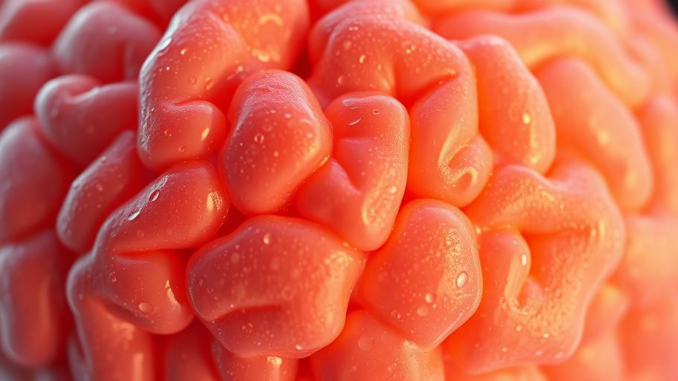
Abstract
Alzheimer’s disease (AD) is a devastating neurodegenerative disorder characterized pathologically by the presence of amyloid plaques and neurofibrillary tangles. While the amyloid hypothesis, which posits that the accumulation of amyloid-beta (Aβ) peptides into plaques is the primary driver of AD pathogenesis, has dominated research and drug development for decades, the clinical trial failures of many Aβ-targeting therapies have spurred re-evaluation of this hypothesis and investigations into alternative and complementary mechanisms. This report provides an in-depth review of amyloid plaque formation, structure, and impact on brain function. It critically examines the amyloid hypothesis and explores the complexities and controversies surrounding it. Furthermore, it discusses the mechanisms of action of Aβ-targeting therapies, their clinical outcomes, and the emerging therapeutic strategies that address other pathological hallmarks of AD and related cellular processes beyond simply targeting amyloid plaques. This includes tau pathology, neuroinflammation, synaptic dysfunction, and metabolic dysregulation. The report emphasizes a holistic view of AD pathogenesis, highlighting the importance of multi-target approaches to effectively combat this complex disease.
Many thanks to our sponsor Esdebe who helped us prepare this research report.
1. Introduction
Alzheimer’s disease (AD) is the most common cause of dementia, affecting millions of people worldwide. The global burden of AD is expected to increase dramatically in the coming decades due to the aging of the population. AD is characterized by progressive cognitive decline, ultimately leading to severe functional impairment and death. The hallmark pathological features of AD are extracellular amyloid plaques composed primarily of aggregated amyloid-beta (Aβ) peptides and intracellular neurofibrillary tangles (NFTs) formed by hyperphosphorylated tau protein.
For many years, the amyloid hypothesis has been the dominant framework for understanding AD pathogenesis [1]. This hypothesis proposes that the accumulation of Aβ peptides, particularly Aβ42, leads to the formation of amyloid plaques, which triggers a cascade of events, including tau phosphorylation, neuroinflammation, synaptic dysfunction, and ultimately neuronal death, leading to cognitive decline. This has led to intense research efforts focused on developing therapies that reduce Aβ production, promote Aβ clearance, or prevent Aβ aggregation.
However, despite decades of research and significant investment in Aβ-targeting therapies, clinical trials have yielded largely disappointing results. While some recent Aβ-targeting antibodies, such as aducanumab and lecanemab, have shown modest clinical benefits in early-stage AD, they are associated with significant adverse effects, including amyloid-related imaging abnormalities (ARIA), and their overall efficacy remains a matter of debate [2, 3]. The limited success of Aβ-targeting therapies has raised questions about the validity of the amyloid hypothesis as the sole driver of AD and has prompted investigations into alternative or complementary mechanisms.
This report will provide a comprehensive overview of amyloid plaques, including their formation, structure, and impact on brain function. It will critically evaluate the amyloid hypothesis, discuss the clinical outcomes of Aβ-targeting therapies, and explore emerging therapeutic strategies that target other pathological hallmarks of AD, as well as related cellular processes, to find a more effective treatment.
Many thanks to our sponsor Esdebe who helped us prepare this research report.
2. Amyloid Plaque Formation and Structure
2.1. Aβ Production and Processing
Aβ peptides are produced through the sequential cleavage of the amyloid precursor protein (APP) by β-secretase (BACE1) and γ-secretase [4]. APP is a transmembrane protein expressed in various tissues, including the brain. The physiological function of APP remains incompletely understood, but it is implicated in neuronal development, synaptic plasticity, and cell adhesion [5].
The initial cleavage of APP by BACE1 generates a soluble APP fragment (sAPPβ) and a membrane-bound C-terminal fragment (CTFβ). CTFβ is then cleaved by γ-secretase, a multi-subunit protease complex, releasing the Aβ peptide and the APP intracellular domain (AICD). γ-secretase cleavage can occur at different sites, resulting in Aβ peptides of varying lengths, with Aβ40 and Aβ42 being the most abundant isoforms. Aβ42 is more hydrophobic and aggregation-prone than Aβ40 and is considered to be more neurotoxic [6].
An alternative processing pathway involves cleavage of APP by α-secretase, which occurs within the Aβ sequence, preventing Aβ formation. α-secretase cleavage generates sAPPα and a membrane-bound C-terminal fragment (CTFα), which is subsequently cleaved by γ-secretase to release AICD and a non-amyloidogenic peptide called p3. Therefore, the balance between α-secretase and β-secretase activity is critical in determining the amount of Aβ produced.
2.2. Aβ Aggregation and Plaque Formation
The aggregation of Aβ peptides into amyloid plaques is a complex process that involves multiple steps, including the formation of oligomers, protofibrils, and fibrils [7]. Aβ oligomers are small, soluble aggregates that are considered to be the most toxic form of Aβ. They can disrupt synaptic function, impair long-term potentiation (LTP), and induce neuronal apoptosis [8]. Protofibrils are larger, elongated aggregates that are precursors to fibrils. Fibrils are insoluble, thread-like structures that are the main component of amyloid plaques.
The process of Aβ aggregation is influenced by several factors, including Aβ concentration, pH, temperature, and the presence of other molecules, such as metal ions and lipids [9]. Metal ions, such as copper, zinc, and iron, can bind to Aβ and promote its aggregation. Lipids, such as cholesterol and gangliosides, can also interact with Aβ and influence its aggregation and toxicity.
Amyloid plaques are typically classified into two main types: diffuse plaques and neuritic plaques. Diffuse plaques are amorphous aggregates of Aβ that lack a dense core and are not associated with significant neuronal damage. Neuritic plaques, on the other hand, are more compact and dense and are surrounded by dystrophic neurites, activated microglia, and reactive astrocytes. Neuritic plaques are considered to be more neurotoxic than diffuse plaques [10]. The structure of neuritic plaques is complex, with a core of aggregated Aβ fibrils surrounded by a halo of activated glial cells and damaged neuronal processes. This surrounding inflammation contributes to the overall neurotoxic effect of the plaque.
2.3. Variations in Aβ Isoforms and Modifications
While Aβ42 is considered the most pathogenic isoform, other Aβ isoforms, such as Aβ40 and Aβ38, also play a role in AD pathogenesis [11]. Aβ40 is the most abundant isoform, but it is less aggregation-prone than Aβ42. Aβ38 is even less aggregation-prone and may even have a protective effect by inhibiting the aggregation of Aβ42.
Aβ peptides can also undergo various post-translational modifications, such as isomerization, racemization, and oxidation. These modifications can alter the aggregation properties and toxicity of Aβ [12]. For example, isomerization of aspartic acid residues in Aβ can increase its aggregation propensity and neurotoxicity. Oxidative modifications, such as methionine oxidation, can also alter the structure and function of Aβ. Furthermore, pyroglutamate-modified Aβ (pE-Aβ) has been shown to be highly toxic and contributes to plaque stability and resistance to degradation [13].
Many thanks to our sponsor Esdebe who helped us prepare this research report.
3. Impact of Amyloid Plaques on Brain Function
3.1. Synaptic Dysfunction
Aβ oligomers have been shown to disrupt synaptic function in various ways [14]. They can interfere with synaptic transmission by inhibiting the release of neurotransmitters, impairing receptor function, and disrupting synaptic plasticity. Aβ oligomers can also induce the loss of synapses, which is a major contributor to cognitive decline in AD. The exact mechanisms by which Aβ oligomers disrupt synaptic function are still being investigated, but several pathways have been implicated, including the activation of kinases, the disruption of calcium homeostasis, and the induction of oxidative stress [15]. The disruption of long-term potentiation (LTP), a cellular mechanism underlying learning and memory, is a key feature of Aβ-induced synaptic dysfunction.
3.2. Neuroinflammation
Amyloid plaques activate microglia and astrocytes, leading to chronic neuroinflammation [16]. Microglia are the resident immune cells of the brain, and they play a critical role in clearing debris and pathogens. However, in AD, microglia become chronically activated by amyloid plaques, releasing pro-inflammatory cytokines and chemokines, such as TNF-α, IL-1β, and IL-6. These inflammatory mediators can exacerbate neuronal damage and contribute to the progression of AD. Reactive astrocytes also contribute to neuroinflammation by releasing inflammatory mediators and by failing to provide trophic support to neurons. The chronic inflammatory response associated with amyloid plaques creates a toxic environment that further promotes neuronal dysfunction and death.
3.3. Neuronal Death
Amyloid plaques can directly and indirectly contribute to neuronal death [17]. Aβ oligomers can directly induce neuronal apoptosis through various mechanisms, including the activation of caspases and the disruption of mitochondrial function. Amyloid plaques can also indirectly contribute to neuronal death by inducing neuroinflammation and oxidative stress, which can damage neurons. Furthermore, the accumulation of Aβ in blood vessels, known as cerebral amyloid angiopathy (CAA), can lead to vascular dysfunction and ischemic damage, further contributing to neuronal loss.
The pattern of neuronal loss in AD is characteristic, with the entorhinal cortex and hippocampus being particularly vulnerable in the early stages of the disease. These brain regions are critical for learning and memory, and their degeneration contributes to the cognitive deficits seen in AD. As the disease progresses, neuronal loss spreads to other brain regions, including the neocortex, leading to more widespread cognitive impairment.
3.4. Impaired Neuronal Network Activity
The presence of amyloid plaques disrupts neuronal network activity, leading to alterations in brain oscillations and connectivity [18]. Studies using electroencephalography (EEG) and magnetoencephalography (MEG) have shown that AD patients have abnormal brain oscillations, including a decrease in alpha and gamma band activity and an increase in slow-wave activity. These changes in brain oscillations are associated with cognitive impairment.
Amyloid plaques can also disrupt the functional connectivity of the brain, leading to alterations in the communication between different brain regions. Studies using functional magnetic resonance imaging (fMRI) have shown that AD patients have decreased functional connectivity in several brain networks, including the default mode network (DMN), which is involved in self-referential thought and mind-wandering. The disruption of neuronal network activity contributes to the cognitive deficits seen in AD.
Many thanks to our sponsor Esdebe who helped us prepare this research report.
4. The Amyloid Hypothesis: Complexities and Controversies
4.1. Evidence Supporting the Amyloid Hypothesis
The amyloid hypothesis is supported by several lines of evidence [19]. Genetic studies have shown that mutations in APP, presenilin 1 (PSEN1), and presenilin 2 (PSEN2) genes, which are involved in Aβ production, cause early-onset familial AD (FAD). These mutations increase Aβ production or alter the ratio of Aβ42 to Aβ40, leading to increased Aβ aggregation. Furthermore, individuals with Down syndrome, who have an extra copy of the APP gene, invariably develop AD pathology at a young age.
Animal studies have also provided strong support for the amyloid hypothesis. Transgenic mice that overexpress mutant human APP develop amyloid plaques and cognitive deficits that resemble those seen in AD. These mice have been used to test Aβ-targeting therapies, and some of these therapies have shown promise in reducing amyloid burden and improving cognitive function in these animal models.
4.2. Challenges to the Amyloid Hypothesis
Despite the evidence supporting the amyloid hypothesis, several challenges and controversies have emerged [20]. One major challenge is the poor correlation between amyloid plaque burden and cognitive decline in some studies. Some individuals with significant amyloid plaque burden do not develop cognitive impairment, while others with relatively low amyloid burden experience significant cognitive decline. This suggests that other factors, such as tau pathology, neuroinflammation, and synaptic dysfunction, may play a more critical role in driving cognitive decline.
The clinical trial failures of many Aβ-targeting therapies have also raised questions about the validity of the amyloid hypothesis. While some Aβ-targeting antibodies, such as aducanumab and lecanemab, have shown modest clinical benefits, the overall effect sizes are small, and they are associated with significant adverse effects. This suggests that targeting Aβ alone may not be sufficient to effectively treat AD. The timing of intervention is also a critical factor, as Aβ-targeting therapies may be more effective in the early stages of the disease, before significant neuronal damage has occurred. The focus has shifted from simply removing plaques to targeting the more toxic soluble oligomers that are believed to initiate the pathological cascade.
4.3. Alternative Theories and the Multifactorial Nature of AD
The limitations of the amyloid hypothesis have led to the development of alternative theories about the causes of AD [21]. The tau hypothesis proposes that tau pathology, rather than amyloid plaques, is the primary driver of cognitive decline in AD. Tau is a microtubule-associated protein that becomes hyperphosphorylated in AD, leading to the formation of NFTs. NFTs disrupt neuronal function and contribute to neuronal death. There is growing evidence that tau pathology is more closely correlated with cognitive decline than amyloid plaque burden.
Other theories propose that neuroinflammation, synaptic dysfunction, mitochondrial dysfunction, and vascular dysfunction play critical roles in AD pathogenesis [22]. It is now widely recognized that AD is a complex, multifactorial disease that involves the interplay of multiple pathological processes. Amyloid plaques, tau pathology, neuroinflammation, and synaptic dysfunction are all interconnected and contribute to the progression of the disease. Therefore, effective therapies for AD may need to target multiple pathological processes simultaneously. For example, neuroinflammation can exacerbate amyloid and tau pathology, creating a positive feedback loop that accelerates disease progression.
Many thanks to our sponsor Esdebe who helped us prepare this research report.
5. Amyloid-Targeting Therapies: Mechanisms and Clinical Outcomes
5.1. Inhibition of Aβ Production
Several therapies have been developed to inhibit Aβ production by targeting β-secretase (BACE1) and γ-secretase [23]. BACE1 inhibitors aim to block the initial cleavage of APP, preventing Aβ formation. γ-secretase inhibitors aim to block the second cleavage of APP, preventing the release of Aβ. However, clinical trials of BACE1 and γ-secretase inhibitors have been largely unsuccessful, with some trials even showing worsening of cognitive function. This may be due to the important physiological functions of BACE1 and γ-secretase, which are involved in the processing of other proteins besides APP. γ-secretase, in particular, is involved in Notch signaling, a critical pathway for cell differentiation and development. Inhibiting γ-secretase can therefore have significant off-target effects. Further research is focused on developing more selective and targeted inhibitors with fewer side effects.
5.2. Enhancement of Aβ Clearance
Another approach to targeting Aβ is to enhance its clearance from the brain [24]. Several therapies have been developed to promote Aβ clearance, including immunotherapy and small molecules that enhance Aβ degradation or transport across the blood-brain barrier. Immunotherapy involves the use of antibodies that bind to Aβ and promote its clearance by microglia or by transport across the blood-brain barrier. Several Aβ-targeting antibodies have been developed, including aducanumab, lecanemab, and donanemab.
Aducanumab was the first Aβ-targeting antibody to be approved by the FDA, but its approval was controversial due to limited evidence of clinical benefit and significant adverse effects, including ARIA. Lecanemab and donanemab have shown more promising results in clinical trials, with modest improvements in cognitive function and reductions in amyloid plaque burden. However, they are also associated with ARIA, which can be serious in some cases. These antibodies selectively target aggregated forms of Aβ, reducing plaque burden more effectively. Enhancing Aβ clearance through other mechanisms, such as stimulating Aβ degradation by enzymes like neprilysin, is also under investigation.
5.3. Inhibition of Aβ Aggregation
Another therapeutic strategy is to inhibit Aβ aggregation, preventing the formation of toxic oligomers and fibrils [25]. Several compounds have been developed to inhibit Aβ aggregation, including small molecules that bind to Aβ and prevent its self-assembly. These compounds can stabilize Aβ monomers, preventing them from aggregating into oligomers and fibrils. Some of these compounds have shown promise in preclinical studies, but their efficacy in clinical trials has been limited. An issue with this approach is that it may not address the underlying cause of Aβ production, and inhibiting aggregation may not be sufficient to prevent the toxic effects of Aβ. Further research is needed to develop more effective aggregation inhibitors.
Many thanks to our sponsor Esdebe who helped us prepare this research report.
6. Emerging Therapeutic Strategies Beyond Amyloid-Targeting
6.1. Tau-Targeting Therapies
Given the strong correlation between tau pathology and cognitive decline, tau-targeting therapies are gaining increasing attention [26]. These therapies aim to reduce tau phosphorylation, prevent tau aggregation, or promote tau clearance. Several tau-targeting antibodies and small molecules are currently in clinical development. Some tau-targeting antibodies aim to block the spread of tau pathology from one neuron to another, while others aim to promote the clearance of aggregated tau. Small molecules are being developed to inhibit tau phosphorylation or prevent tau aggregation. Early clinical trials have shown some promise, but further research is needed to determine the efficacy of tau-targeting therapies. The development of tau PET tracers has greatly aided in monitoring the effects of these therapies.
6.2. Targeting Neuroinflammation
Neuroinflammation plays a critical role in AD pathogenesis, and targeting neuroinflammation is a promising therapeutic strategy [27]. Several anti-inflammatory agents are being investigated as potential treatments for AD, including non-steroidal anti-inflammatory drugs (NSAIDs), COX-2 inhibitors, and inhibitors of inflammatory cytokines. However, clinical trials of NSAIDs and COX-2 inhibitors have been largely unsuccessful, possibly because these agents have broad effects and may not specifically target the inflammatory pathways involved in AD. More targeted approaches, such as inhibitors of specific inflammatory cytokines or chemokines, are being developed. Modulating microglial activity, shifting them from a pro-inflammatory to a neuroprotective phenotype, is also being explored. Diet and lifestyle interventions, such as the Mediterranean diet, are also being investigated for their potential anti-inflammatory effects.
6.3. Enhancing Synaptic Function
Synaptic dysfunction is a major contributor to cognitive decline in AD, and enhancing synaptic function is a key therapeutic goal [28]. Several approaches are being investigated to enhance synaptic function, including increasing neurotransmitter levels, promoting synaptic plasticity, and protecting synapses from damage. Cholinesterase inhibitors, which increase acetylcholine levels in the brain, are currently the only approved symptomatic treatments for AD. However, their efficacy is limited, and they do not address the underlying cause of the disease. Other approaches to enhancing synaptic function include the use of neurotrophic factors, such as brain-derived neurotrophic factor (BDNF), and the development of drugs that promote synaptic plasticity. Targeting specific synaptic receptors, such as NMDA receptors, to enhance synaptic transmission is also being explored. Lifestyle interventions, such as exercise and cognitive training, can also enhance synaptic function and improve cognitive performance.
6.4. Addressing Metabolic Dysfunction
Metabolic dysfunction, including insulin resistance and impaired glucose metabolism, is increasingly recognized as a risk factor for AD [29]. Addressing metabolic dysfunction is a promising therapeutic strategy. Several approaches are being investigated, including the use of insulin-sensitizing drugs, such as metformin, and the development of ketogenic diets. Ketogenic diets, which are high in fat and low in carbohydrates, can provide an alternative energy source for the brain and improve cognitive function in AD patients. Clinical trials are underway to evaluate the efficacy of these interventions. Lifestyle interventions, such as exercise and diet, can also improve metabolic function and reduce the risk of AD. The role of the gut microbiome in metabolic dysfunction and AD is also being investigated.
6.5. Combination Therapies and Personalized Medicine
Given the multifactorial nature of AD, it is likely that combination therapies that target multiple pathological processes will be more effective than single-target therapies [30]. Combination therapies could include Aβ-targeting agents, tau-targeting agents, anti-inflammatory agents, and drugs that enhance synaptic function or address metabolic dysfunction. Personalized medicine approaches, which tailor treatment to the individual patient based on their genetic profile, biomarker profile, and other clinical characteristics, may also improve the efficacy of AD therapies. Biomarkers, such as Aβ and tau levels in cerebrospinal fluid (CSF) and PET imaging, can help identify patients who are most likely to benefit from specific therapies. Genetic testing can identify individuals who are at increased risk of developing AD and may benefit from early intervention strategies. The future of AD treatment likely lies in a combination of multi-target therapies and personalized approaches.
Many thanks to our sponsor Esdebe who helped us prepare this research report.
7. Conclusion
Amyloid plaques are a key pathological feature of Alzheimer’s disease and have been the primary focus of research and drug development for decades. While the amyloid hypothesis has provided a valuable framework for understanding AD pathogenesis, the clinical trial failures of many Aβ-targeting therapies have highlighted the complexities of the disease and the need for a more holistic view. Emerging therapeutic strategies that target other pathological hallmarks of AD, such as tau pathology, neuroinflammation, synaptic dysfunction, and metabolic dysregulation, are showing promise. Combination therapies and personalized medicine approaches may be necessary to effectively combat this complex disease. The future of AD research lies in a better understanding of the interplay between different pathological processes and the development of multi-target therapies that address the multifactorial nature of the disease.
Many thanks to our sponsor Esdebe who helped us prepare this research report.
References
[1] Hardy, J., & Selkoe, D. J. (2002). The amyloid hypothesis of Alzheimer’s disease: progress and problems on the road to therapeutics. Science, 297(5580), 353-356.
[2] van Dyck, C. H., Swanson, C. J., Aisen, P., Bateman, R. J., Chen, C., Gee, M., … & Sandrock, A. (2023). Lecanemab in early Alzheimer’s disease. New England Journal of Medicine, 388(1), 9-21.
[3] Mullard, A. (2021). FDA approves controversial Alzheimer’s drug. Nature Reviews Drug Discovery, 20(7), 491-492.
[4] De Strooper, B., Vassar, R., & Golde, T. (2010). The secretases: enzymes with therapeutic potential in Alzheimer disease. Nature Reviews Neurology, 6(2), 99-107.
[5] Müller, U. C., & Zheng, H. (2012). Physiological functions of APP family proteins. Cold Spring Harbor Perspectives in Medicine, 2(7), a006288.
[6] Jarrett, J. T., Berger, E. P., & Lansbury Jr, P. T. (1993). The carboxy terminus of the beta-amyloid protein is critical for the seeding of amyloid formation: implications for the pathogenesis of Alzheimer’s disease. Biochemistry, 32(18), 4693-4697.
[7] Knowles, T. P., Vendruscolo, M., & Dobson, C. M. (2014). The amyloid state and its association with protein misfolding diseases. Nature Reviews Molecular Cell Biology, 15(6), 384-396.
[8] Selkoe, D. J. (2008). Soluble oligomers of amyloid beta-protein and its precursors impair synaptic plasticity and behavior. Cold Spring Harbor Perspectives in Biology, 2(7), a000317.
[9] Makin, O. S. (2005). Microscopic analysis of the structure, morphology and aggregation of amyloid fibrils. Current Opinion in Structural Biology, 15(5), 571-578.
[10] Serrano-Pozo, A., Frosch, M. P., Bachhuber, A. G., McLean, T. X., Betensky, R. A., Hyman, B. T., & Growdon, J. H. (2011). Neuropathological substrates of aging and Alzheimer’s disease. Annals of the New York Academy of Sciences, 1229(1), 119-135.
[11] Wiltfang, J., Esselmann, H., Bibl, M., Smidt, J., Hulstaert, F., & Rüther, E. (2002). Amyloid beta peptide ratio 42/40 in cerebrospinal fluid differentiates Alzheimer’s disease from normal aging. Biological Psychiatry, 52(6), 555-562.
[12] D’Adamio, L., & Tagliavini, F. (2000). Isomerization and racemization of aspartyl residues in amyloidogenic peptides: pathological implications. Amyloid, 7(1), 1-12.
[13] Schilling, S., Miners, J. S., & Ovádi, J. (2021). Pyroglutamate-Aβ: A seed for pathological Aβ aggregation in Alzheimer’s disease. Journal of Neurochemistry, 156(4), 418-437.
[14] Mucke, L., & Selkoe, D. J. (2012). Neurotoxicity of amyloid β-protein: synaptic and network dysfunction. Neuron, 78(5), 403-423.
[15] Ferreira, S. T., Clarke, J. R., Bomfim, T. R., & De Felice, F. G. (2015). Insulin resistance in Alzheimer’s disease. Nature Reviews Neuroscience, 15(11), 676-694.
[16] Heneka, M. T., Golenbock, D. T., Latz, E., Morgan, D., Brown, R., Halle, A., … & Weber, F. E. (2015). The inflammasome in neurodegenerative diseases. Nature, 493(7434), 674-678.
[17] Mattson, M. P. (2004). Pathways towards and away from Alzheimer’s disease. Nature, 430(7000), 631-639.
[18] Palop, J. J., & Mucke, L. (2010). Amyloid-β-induced neuronal dysfunction in Alzheimer’s disease: from synapses toward neural networks. Nature Neuroscience, 13(7), 812-818.
[19] Glenner, G. G., & Wong, C. W. (1984). Alzheimer’s disease: initial report of the purification and characterization of a novel cerebrovascular amyloid protein. Biochemical and Biophysical Research Communications, 120(3), 885-890.
[20] Herrup, K. (2015). The amyloid hypothesis: an evaluation and appraisal. Journal of Neurochemistry, 93(5), 1062-1073.
[21] Mudher, A., & Lovestone, S. (2002). Alzheimer’s disease-do tauists and beta-amylonists need to get together?. Trends in Neurosciences, 25(1), 22-26.
[22] Jack Jr, C. R., Holtzman, D. M., Amariglio, R. E., Knopman, D. S., Trojanowski, J. Q., Petersen, R. C., … & Albert, M. S. (2018). Update on hypothetical model of Alzheimer’s disease biomarkers. The Lancet Neurology, 12(2), 207-216.
[23] Vassar, R. (2014). BACE1 enzyme as a drug target for Alzheimer’s disease. Alzheimer’s Research & Therapy, 6(9), 89.
[24] Schenk, D., Barbour, R., Dunn, W., Gordon, G., Grajeda, H., Guido, T., … & Lieberburg, I. (1999). Immunization with amyloid-β attenuates Alzheimer-disease-like pathology in the PDAPP mouse. Nature, 400(6740), 173-177.
[25] Tjernberg, L. O., Näslund, J., Lindqvist, F., Johansson, J., Karlström, A. R., Thyberg, J., … & Nordstedt, C. (1996). Arrest of β-amyloid fibril formation by a pentapeptide segment of human apolipoprotein E. Journal of Biological Chemistry, 271(15), 8545-8548.
[26] Iqbal, K., Grundke-Iqbal, I., Smith, A. J., George, L., Tung, Y. C., & Zaidi, T. (2010). Defective brain glucose metabolism is associated with a progressive accumulation of abnormally phosphorylated tau. Acta Neuropathologica, 121(1), 67-77.
[27] Akiyama, H., Barger, S., Barnum, S., Bradt, B., Bauer, J., Cole, G. M., … & McGeer, P. L. (2000). Inflammation and Alzheimer’s disease. Neurobiology of Aging, 21(3), 383-421.
[28] Terry, R. D., Masliah, E., Salmon, D. P., Butters, N., DeTeresa, R., Hill, R., … & Katzman, R. (1991). Physical basis of cognitive alterations in Alzheimer’s disease: synapse loss is the major correlate of cognitive impairment. Annals of Neurology, 30(4), 572-580.
[29] de la Monte, S. M., & Wands, J. R. (2008). Alzheimer’s disease is type 3 diabetes-evidence reviewed. Journal of Diabetes Science and Technology, 2(6), 1101-1113.
[30] Cummings, J. L. (2011). Combining therapies for Alzheimer’s disease. Alzheimer’s Research & Therapy, 3(5), 25.
So, if amyloid plaques are like uninvited guests at a party, and clearing them out doesn’t guarantee everyone suddenly starts having a good time, are we sure we’re not just obsessing over decorations while the real problem is the awful music (or, you know, neuroinflammation)?
That’s a fantastic analogy! The ‘awful music’ (neuroinflammation) is definitely getting more attention as a key player. It’s increasingly clear that a multi-pronged approach, tackling both amyloid plaques *and* the inflammatory response, is essential for effective treatments. Thanks for highlighting this crucial aspect!
Editor: MedTechNews.Uk
Thank you to our Sponsor Esdebe
So, we’re thinking plaques might be more like barnacles on a ship, slowing things down but not necessarily sinking it? Perhaps we should focus on the pirates (tau tangles?) or the rough seas (inflammation) actually causing the damage?
That’s a great way to put it! The barnacle analogy is very insightful. It’s becoming increasingly clear that while amyloid plaques play a role, the tau tangles and inflammation are major drivers of the disease. Exploring treatments that target these factors offers great promise for tackling Alzheimer’s from multiple angles. Thanks for the comment!
Editor: MedTechNews.Uk
Thank you to our Sponsor Esdebe
Given the disappointing outcomes of solely targeting amyloid plaques, how might combination therapies addressing both amyloid and tau pathologies, along with neuroinflammation, offer a more comprehensive and effective approach to slowing disease progression?
That’s a great question! Indeed, the idea of a multi-pronged approach is gaining traction. By targeting amyloid plaques, tau tangles, and neuroinflammation simultaneously, we might be able to achieve a synergistic effect, addressing the disease from multiple angles and potentially slowing its progression more effectively. Combination therapies could be the key to unlocking more successful treatments.
Editor: MedTechNews.Uk
Thank you to our Sponsor Esdebe