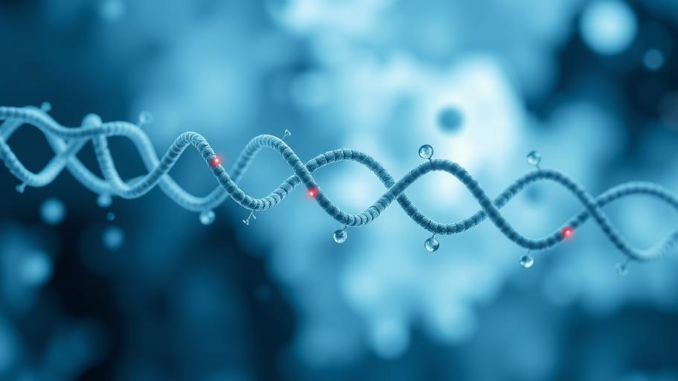
Antisense Oligonucleotides: A Comprehensive Review of Mechanisms, Modifications, Delivery Strategies, and Therapeutic Applications Beyond Neurology
Abstract
Antisense oligonucleotides (ASOs) represent a powerful class of therapeutic agents capable of modulating gene expression with remarkable specificity. This review provides a comprehensive overview of ASO technology, extending beyond the well-documented neurological applications to encompass a broader spectrum of therapeutic areas. We delve into the intricate mechanisms of ASO action, including RNase H-mediated degradation, steric hindrance of translation, and alternative splicing modulation. A critical evaluation of chemical modifications designed to enhance ASO stability, delivery, and efficacy is presented, encompassing phosphorothioate backbones, sugar modifications (e.g., 2′-O-methyl, 2′-O-methoxyethyl), and base modifications. While delivery to the central nervous system (CNS) poses specific challenges, we explore both established (e.g., intrathecal administration) and emerging strategies (e.g., enhanced delivery utilizing viral vectors) for systemic and tissue-specific ASO delivery. The inherent risk of off-target effects necessitates meticulous design and preclinical evaluation, and we discuss current strategies to mitigate these risks, including sequence optimization and chemical modification approaches. Regulatory considerations for ASO-based therapies are addressed, highlighting the evolving landscape for accelerated approval pathways. Beyond the clinical successes in spinal muscular atrophy (SMA), Huntington’s disease, and Alzheimer’s disease, we examine the expanding therapeutic potential of ASOs in oncology, cardiovascular disease, and rare genetic disorders. Finally, we analyze current market trends, emerging technologies, and future perspectives that will shape the trajectory of ASO therapeutics in the coming years.
1. Introduction
Antisense oligonucleotide (ASO) technology has emerged as a transformative modality in drug discovery and development. These synthetic, single-stranded DNA or RNA molecules are designed to bind to specific messenger RNA (mRNA) sequences through Watson-Crick base pairing, thereby modulating gene expression. The fundamental principle underlying ASO action is the ability to selectively target and manipulate the expression of disease-causing genes. This targeted approach offers a distinct advantage over traditional small-molecule drugs, which often interact with multiple targets and can lead to off-target effects and toxicity. While initial enthusiasm for ASO therapeutics was tempered by challenges related to stability, delivery, and immunogenicity, significant advancements in chemical modifications and delivery strategies have propelled ASOs to the forefront of precision medicine. The approval of several ASO-based therapies for a range of diseases, including spinal muscular atrophy (SMA) [1], familial amyloid polyneuropathy (FAP) [2], and Duchenne muscular dystrophy (DMD) [3], has validated the potential of this technology. This review aims to provide an in-depth exploration of ASO mechanisms of action, chemical modifications, delivery strategies, off-target effects, regulatory considerations, and clinical applications, extending beyond the prominent neurological applications to highlight the broader therapeutic landscape.
2. Mechanisms of ASO Action
The therapeutic efficacy of ASOs hinges on their ability to modulate gene expression through various mechanisms. The predominant mechanisms include:
2.1 RNase H-Mediated mRNA Degradation
RNase H is an endogenous enzyme that specifically recognizes and cleaves RNA in DNA:RNA heteroduplexes [4]. ASOs designed to elicit RNase H activity typically contain a DNA gap region flanked by modified nucleotides. Upon binding to the target mRNA, the DNA gap region forms a DNA:RNA heteroduplex, which is recognized by RNase H. The enzyme then cleaves the mRNA strand within the heteroduplex, leading to its degradation and subsequent reduction in protein expression [5]. The design of ASOs that effectively recruit RNase H requires careful consideration of the DNA gap length and the flanking modification patterns. Too short a gap may not recruit RNase H effectively, while a long gap may reduce binding affinity to the target mRNA. Phosphorothioate (PS) modifications, discussed later, are crucial for RNase H recruitment and protection from nuclease degradation.
2.2 Steric Blocking of Translation
ASOs can also inhibit gene expression by sterically blocking the ribosome from binding to the mRNA, thereby preventing translation of the mRNA into protein [6]. This mechanism typically requires ASOs to bind to the 5′ untranslated region (UTR) or the start codon region of the mRNA. Steric blocking is independent of RNase H and is often employed when mRNA degradation is not desired, such as in splice switching applications. ASOs designed for steric blocking often incorporate 2′-O-methyl (2′-OMe) or 2′-O-methoxyethyl (2′-MOE) modifications, which enhance binding affinity and stability.
2.3 Modulation of RNA Splicing
Alternative splicing is a crucial regulatory mechanism that allows a single gene to produce multiple protein isoforms. ASOs can be designed to target specific splice sites or regulatory elements within the pre-mRNA, thereby altering the splicing pattern and modulating the production of different protein isoforms [7]. This mechanism is particularly relevant for treating diseases caused by aberrant splicing, such as spinal muscular atrophy (SMA). Nusinersen (Spinraza®), an ASO approved for SMA treatment, works by altering the splicing of the SMN2 gene to increase the production of functional SMN protein [1]. The design of splice-switching ASOs requires precise targeting of specific splicing elements and careful consideration of the potential for off-target splicing effects.
2.4 MicroRNA (miRNA) Inhibition
MicroRNAs (miRNAs) are small non-coding RNA molecules that regulate gene expression by binding to the 3′ UTR of target mRNAs, leading to translational repression or mRNA degradation [8]. ASOs, often referred to as antagomirs or miRNA inhibitors, can be designed to bind to specific miRNAs, thereby preventing them from binding to their target mRNAs and restoring the expression of the target genes. This mechanism is particularly relevant for treating diseases where miRNAs are dysregulated, such as cancer and cardiovascular disease [9].
3. Chemical Modifications for Enhanced ASO Properties
Native DNA and RNA oligonucleotides are susceptible to degradation by nucleases and exhibit poor cellular uptake. Chemical modifications are crucial for enhancing the stability, delivery, and efficacy of ASOs. Key modifications include:
3.1 Phosphorothioate (PS) Backbone
Replacing one of the non-bridging oxygen atoms in the phosphodiester backbone with a sulfur atom creates a phosphorothioate (PS) linkage. This modification significantly enhances the nuclease resistance of ASOs, prolonging their half-life in vivo [10]. PS modifications also increase the binding affinity of ASOs to serum proteins, which can improve their distribution and cellular uptake. However, PS modifications can also increase the risk of off-target effects and immunogenicity. The PS modification can be either Rp or Sp diastereoisomers, both of which will be present in a PS modified ASO. Research suggests that these diastereoisomers can exhibit different pharmacological properties, but strategies for producing stereopure PS ASOs are challenging and currently not in widespread use.
3.2 Sugar Modifications (2′-O-Methyl, 2′-O-Methoxyethyl, Constrained Ethyl)
Modifications at the 2′ position of the ribose sugar can significantly improve the binding affinity and stability of ASOs. 2′-O-methyl (2′-OMe) and 2′-O-methoxyethyl (2′-MOE) modifications are commonly used to enhance the binding affinity of ASOs to their target mRNAs and to increase their resistance to nuclease degradation [11]. Constrained ethyl (cEt) modifications provide even greater binding affinity and nuclease resistance compared to 2′-OMe and 2′-MOE modifications [12]. These modifications can also reduce the immunogenicity of ASOs.
3.3 Base Modifications
Modifications to the nucleobases (adenine, guanine, cytosine, thymine, uracil) can further enhance the properties of ASOs. For example, 5-methylcytosine (5-MeC) modifications can increase the binding affinity of ASOs to their target mRNAs and reduce their immunogenicity [13]. Locked nucleic acids (LNAs) are modified nucleosides with a methylene bridge connecting the 2′-O and 4′-C atoms of the ribose sugar, resulting in a constrained conformation and exceptionally high binding affinity to complementary RNA sequences [14].
3.4 Conjugation Strategies
Conjugation of ASOs to various molecules, such as lipids, peptides, or antibodies, can improve their delivery to specific tissues or cells. GalNAc (N-acetylgalactosamine) conjugation, for example, is used to target ASOs to hepatocytes via the asialoglycoprotein receptor, enhancing their delivery to the liver [15]. Peptide conjugation can facilitate cellular uptake via receptor-mediated endocytosis. Antibody conjugation can target ASOs to specific cell types expressing the corresponding antigen.
The optimal combination of chemical modifications depends on the specific therapeutic application and the target tissue. Balancing stability, binding affinity, delivery efficiency, and the potential for off-target effects is crucial for the successful development of ASO-based therapies. The use of gapmers where the central region is unmodified DNA flanked by modified nucleotide regions such as MOE is a common strategy to allow RNase H mediated degradation of the target mRNA.
4. Delivery Strategies for ASOs
Effective delivery of ASOs to the target tissue and cells is a critical determinant of their therapeutic efficacy. Delivery challenges vary depending on the target tissue and the route of administration. Systemic delivery faces challenges related to serum protein binding, nuclease degradation, and limited cellular uptake.
4.1 Local Delivery
Local delivery approaches, such as intrathecal administration for CNS targets, can bypass systemic barriers and achieve high concentrations of ASOs in the target tissue. Intrathecal administration involves injecting ASOs directly into the cerebrospinal fluid (CSF), allowing them to distribute throughout the CNS [16]. This route of administration is commonly used for treating neurological disorders, such as SMA and Huntington’s disease. Other local delivery routes include topical administration for skin disorders and intravitreal injection for eye diseases.
4.2 Systemic Delivery
Systemic delivery requires ASOs to overcome several barriers, including serum protein binding, nuclease degradation, and limited cellular uptake. Chemical modifications, such as PS modifications and sugar modifications, can improve the stability and serum protein binding of ASOs. Conjugation strategies, such as GalNAc conjugation, can enhance the delivery of ASOs to specific tissues, such as the liver [15]. Liposomes and nanoparticles can also be used to encapsulate ASOs and protect them from degradation, facilitating their delivery to target cells [17]. Furthermore, viral vectors are being explored as a means to deliver ASOs to specific cell types, offering the potential for long-term gene silencing.
4.3 Targeted Delivery Strategies
Targeted delivery strategies aim to deliver ASOs specifically to the target cells or tissues, minimizing off-target effects and maximizing therapeutic efficacy. Antibody-mediated delivery involves conjugating ASOs to antibodies that recognize specific cell surface antigens, allowing for targeted delivery to cells expressing the antigen [18]. Aptamers, which are short single-stranded DNA or RNA molecules that bind to specific target molecules with high affinity, can also be used to deliver ASOs to specific cells or tissues [19]. Extracellular vesicles (EVs), such as exosomes, are naturally occurring nanoparticles that can be engineered to deliver ASOs to specific cells or tissues [20].
5. Off-Target Effects and Mitigation Strategies
Off-target effects are a significant concern for ASO-based therapies. These effects can arise from the binding of ASOs to unintended mRNA targets or from interactions with proteins or other cellular components. Off-target binding to unintended mRNA targets can lead to unintended modulation of gene expression, resulting in adverse effects [21]. Interactions with proteins or other cellular components can trigger immune responses or other toxicities.
5.1 Sequence Optimization
Careful sequence design is crucial for minimizing off-target effects. Bioinformatic tools can be used to predict potential off-target binding sites based on sequence homology. Selecting ASO sequences with minimal homology to other genes can reduce the risk of off-target binding.
5.2 Chemical Modifications
Certain chemical modifications can reduce the risk of off-target effects. For example, incorporating modified nucleotides that increase the binding affinity of ASOs to their target mRNA can reduce the concentration of ASOs required for therapeutic efficacy, thereby minimizing the potential for off-target binding [22].
5.3 Preclinical Evaluation
Thorough preclinical evaluation is essential for identifying and characterizing potential off-target effects. In vitro studies can be used to assess the binding of ASOs to unintended mRNA targets. In vivo studies can be used to assess the toxicity and immunogenicity of ASOs. RNA sequencing (RNA-Seq) can be used to identify unintended changes in gene expression caused by ASOs.
5.4 Target Validation
Ensuring that the intended mRNA target is the correct target for modulating the disease is crucial. It is essential to establish that modulating the target has the desired effect on disease pathology. This can be achieved with genetic knockout or knockdown experiments and confirmed through multiple independent methods. If the chosen target is wrong this will of course lead to off target effects, but the intended target is simply incorrect.
6. Regulatory Landscape for ASO-Based Therapies
The regulatory landscape for ASO-based therapies is evolving. Regulatory agencies, such as the FDA in the United States and the EMA in Europe, have established guidelines for the development and approval of ASO-based therapies [23]. These guidelines address various aspects of ASO development, including preclinical studies, clinical trials, manufacturing, and post-market surveillance. The FDA has granted accelerated approval to several ASO-based therapies based on surrogate endpoints, such as changes in protein expression levels, recognizing the potential for ASOs to address unmet medical needs [24]. The EMA has also established adaptive pathways for the approval of ASO-based therapies, allowing for early access to promising treatments for patients with serious diseases. The regulatory environment is becoming increasingly favorable for ASO-based therapies as the technology matures and more clinical data become available.
7. Clinical Development of ASOs
ASOs have demonstrated clinical efficacy in a range of diseases, including neurological disorders, genetic diseases, and cancer. Several ASO-based therapies have been approved by regulatory agencies, and numerous clinical trials are ongoing to evaluate the potential of ASOs for treating other diseases. Nusinersen (Spinraza®), an ASO approved for SMA treatment, has revolutionized the treatment of this devastating disease [1]. Inotersen (Tegsedi®), an ASO approved for FAP treatment, has demonstrated significant clinical benefit in patients with this rare genetic disorder [2]. Eteplirsen (Exondys 51®), an ASO approved for DMD treatment, has shown modest clinical benefit in some patients with this progressive muscle-wasting disease [3]. Volanesorsen (Waylivra®), an ASO approved for familial chylomicronemia syndrome (FCS), reduces triglyceride levels in patients with this rare metabolic disorder [25]. Clinical trials are ongoing to evaluate the potential of ASOs for treating other neurological disorders, such as Huntington’s disease and Alzheimer’s disease. Clinical trials are also evaluating the potential of ASOs for treating various types of cancer, cardiovascular disease, and other diseases.
8. Market Trends and Future Perspectives
The market for ASO-based therapies is growing rapidly, driven by the increasing clinical validation of ASO technology and the growing number of approved ASO-based therapies. The global ASO therapeutics market is expected to reach billions of dollars in the coming years [26]. Several factors are driving the growth of the ASO therapeutics market, including the increasing prevalence of genetic diseases, the growing demand for personalized medicine, and the increasing investment in ASO research and development. Emerging technologies, such as CRISPR-Cas9 gene editing and RNA interference (RNAi), are complementary to ASO technology and may be used in combination with ASOs to achieve more potent and durable therapeutic effects. Future perspectives for ASO therapeutics include the development of more targeted delivery strategies, the development of ASOs with improved efficacy and safety profiles, and the expansion of ASO applications to treat a wider range of diseases. Improved manufacturing processes to produce ASOs at large scale at low cost are also important, particularly for addressing unmet medical needs in developing countries.
9. Conclusion
Antisense oligonucleotide (ASO) technology has emerged as a powerful and versatile platform for modulating gene expression and treating a wide range of diseases. Significant advancements in chemical modifications, delivery strategies, and preclinical evaluation have propelled ASOs to the forefront of precision medicine. The approval of several ASO-based therapies for neurological disorders, genetic diseases, and other conditions has validated the potential of this technology. As the market for ASO-based therapies continues to grow, future research efforts will focus on developing more targeted delivery strategies, improving the efficacy and safety profiles of ASOs, and expanding ASO applications to treat a wider range of diseases. ASOs represent a promising avenue for developing novel therapeutics that can address unmet medical needs and improve the lives of patients worldwide. While neurological applications have been at the forefront, the expanded exploration of ASOs in areas such as oncology, cardiovascular disease, and rare genetic disorders holds immense promise for the future of precision medicine. Understanding the mechanisms of action, optimizing chemical modifications, and refining delivery strategies remain critical for realizing the full potential of ASO therapeutics.
References
[1] Finkel, R. S., Chiriboga, C. A., Vajsar, J., Day, J. W., Montes, J., De Vivo, D. C., … & Bennett, C. F. (2016). Treatment of infantile-onset spinal muscular atrophy with nusinersen: a phase 2, open-label, dose-escalation study. The Lancet, 388(10063), 3017-3026.
[2] Benson, M. D., Waddington-Cruz, M., Berk, J. L., Polydefkis, M., Dyck, P. J., Wang, A. K., … & Ackermann, E. J. (2018). Inotersen treatment for patients with hereditary transthyretin amyloidosis. New England Journal of Medicine, 379(1), 22-31.
[3] Mendell, J. R., Goemans, N. M., Lowes, L. P., Alfano, L. N., Berry, K., Bushby, K., … & Eteplirsen Study Group. (2013). Eteplirsen for the treatment of Duchenne muscular dystrophy. Annals of neurology, 74(5), 637-647.
[4] Crooke, S. T., & Bennett, C. F. (2019). Antisense technology: an overview. Antisense drug technology: principles, strategies, and applications, 1-38.
[5] Bennett, C. F., & Swayze, E. E. (2010). RNA targeting therapeutics: molecular mechanisms of antisense oligonucleotides as a therapeutic platform. Annual review of pharmacology and toxicology, 50, 259-293.
[6] Kole, R., Krainer, A. R., & Altman, S. (2012). RNA therapeutics: beyond RNA interference and antisense oligonucleotides. Nature Reviews Drug Discovery, 11(2), 125-140.
[7] Havens, M. A., Duelli, D. M., & Hastings, M. L. (2013). Targeting RNA splicing for disease therapy. Wiley Interdisciplinary Reviews: RNA, 4(3), 247-261.
[8] Bartel, D. P. (2004). MicroRNAs: genomics, biogenesis, mechanism, and function. Cell, 116(2), 281-297.
[9] Rupaimoole, R., & Slack, F. J. (2017). MicroRNA therapeutics: towards a new era for the management of cancer and other diseases. Nature Reviews Drug Discovery, 16(3), 203-222.
[10] Eckstein, F. (2014). Phosphorothioate oligodeoxynucleotides: an interesting class of synthetic DNA. Antisense Nucleic Acid Drug Development, 10(2), 117-121.
[11] Freier, S. M., & Altmann, K. H. (1997). The ups and downs: Chemical modifications applied to oligonucleotide therapeutics. Nucleic Acids Research, 25(22), 4429-4443.
[12] Seth, P. P., Siwkowski, A. M., Allerson, C. R., Vasquez, G., Lee, S., Prakash, T. P., … & Crooke, S. T. (2009). Synthesis and biophysical evaluation of 2′-constrained ethyl and 2′-O-methyl oligomers. Journal of organic chemistry, 74(11), 4141-4151.
[13] Sanghvi, Y. S. (1993). Heterocyclic base modifications in nucleic acids and their applications in antisense oligodeoxynucleotides. Antisense research and development, 3(1), 37-43.
[14] Koshkin, A. A., Singh, S. K., Nielsen, P., Vester, B., & Wengel, J. (1998). LNA (Locked Nucleic Acid): synthesis of the 2′-O-aminoethyl-LNA analogue. Tetrahedron letters, 39(23), 4381-4384.
[15] Nair, J. K., Willoughby, J. L., Chan, A., Charisse, K., Alam, M. R., Wang, Q., … & Fitzgerald, K. (2014). Multivalent N-acetylgalactosamine-conjugated siRNA localizes selectively to the liver and achieves efficient gene silencing. Journal of the American Chemical Society, 136(49), 16958-16961.
[16] Rinaldi, C., & Wood, M. J. A. (2018). Antisense oligonucleotides: the next frontier for treatment of neurological disorders. Brain, 141(7), 1866-1890.
[17] Juliano, R. L. (2016). Nanoparticles for delivery of antisense oligonucleotides. Advances in genetics, 96, 109-144.
[18] Song, E., Zhu, P., Lee, J. S. H., Chowdhury, D., Kussman, S., Dykxhoorn, D. M., … & Lieberman, J. (2005). Antibody mediated in vivo delivery of small interfering RNAs targeting Fas protects mice from fulminant hepatitis. Nature biotechnology, 23(6), 709-717.
[19] Zhou, J., Rossi, J. J., & Maher III, L. J. (2012). Aptamers as targeted therapeutics: current potential and challenges. Nature Reviews Drug Discovery, 11(3), 181-202.
[20] El Andaloussi, S., Mäger, I., Breakefield, X. O., & Wood, M. J. (2013). Extracellular vesicles: biology and emerging therapeutic opportunities. Nature Reviews Drug Discovery, 12(5), 347-357.
[21] Geary, R. S. (2016). Clinical development of antisense oligonucleotides: state of the art. Current opinion in pharmacology, 28, 66-73.
[22] Crooke, S. T., Wang, S., Vickers, T. A., & Shen, W. (2018). Cellular uptake and trafficking of antisense oligonucleotides. Nature biotechnology, 36(9), 864-874.
[23] Committee for Medicinal Products for Human Use (CHMP). (2018). Guideline on similar medicinal products containing biotechnology-derived proteins as active substance: non-clinical and clinical issues. European Medicines Agency.
[24] U.S. Food and Drug Administration (FDA). (2018). Expedited Programs for Serious Conditions – Drugs and Biologics. U.S. Department of Health and Human Services.
[25] Witztum, J. L., Gaudet, D., Freedman, S. D., Alexander, V. J., Digenan, G. G., Williams, K. R., … & Hughes, S. G. (2019). Volanesorsen and triglyceride levels in familial chylomicronemia syndrome. New England Journal of Medicine, 381(6), 531-542.
[26] Global Market Insights. (2023). Antisense & RNAi Therapeutics Market Size by Technology (Antisense Oligonucleotides [ASO], RNA Interference [RNAi]), By Route of Administration, By Therapeutic Area, Industry Analysis Report, Regional Outlook, Application Potential, Competitive Market Share & Forecast, 2023 – 2032.
Given the diverse range of chemical modifications discussed, are there predictive models available to assess the impact of specific modifications on off-target binding affinity and potential toxicity *before* synthesis, rather than relying solely on post-synthesis evaluation?
That’s a great question! Predictive models are definitely an area of active development. While no model is perfect, machine learning algorithms are increasingly being used to predict off-target binding and potential toxicity based on chemical structure and sequence. Continued refinement and validation of these models are crucial for accelerating ASO development. Thanks for bringing this up!
Editor: MedTechNews.Uk
Thank you to our Sponsor Esdebe