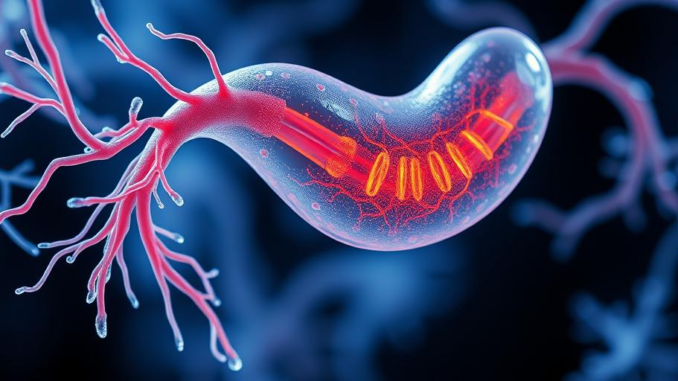
Bioengineered Pancreas: A Comprehensive Review of Bioprinting, Immunological Challenges, and Clinical Translation
Many thanks to our sponsor Esdebe who helped us prepare this research report.
Abstract
Type 1 diabetes (T1D) is an autoimmune disease characterized by the destruction of insulin-producing pancreatic beta cells, leading to lifelong dependence on exogenous insulin. While insulin therapy and continuous glucose monitoring (CGM) systems have improved glycemic control, they fall short of replicating the intricate feedback mechanisms of a healthy pancreas. This review provides a comprehensive overview of the burgeoning field of bioengineered pancreas development, with a particular focus on 3D bioprinting as a promising avenue for creating functional islet grafts. We examine the various bioprinting techniques employed, highlight the challenges and opportunities associated with different cell sources (including stem cells and primary islet cells), and delve into the critical aspects of vascularization and immune modulation. Furthermore, we explore the regulatory landscape, ethical considerations, and the potential market for bioengineered pancreas technologies, emphasizing the need for rigorous preclinical and clinical evaluation to ensure safety and efficacy. We conclude by outlining future directions for research, focusing on personalized approaches and advancements in biomaterials and immunoprotection strategies to facilitate the successful translation of bioengineered pancreata to clinical application.
Many thanks to our sponsor Esdebe who helped us prepare this research report.
1. Introduction
Type 1 diabetes mellitus (T1D) is a chronic autoimmune disease resulting in the selective destruction of insulin-producing beta cells within the pancreatic islets of Langerhans. This loss of functional beta cells leads to absolute insulin deficiency, necessitating lifelong exogenous insulin administration to maintain glucose homeostasis [1]. While advancements in insulin analogs, delivery devices, and continuous glucose monitoring (CGM) systems have significantly improved the management of T1D, these therapies often fail to fully replicate the dynamic and nuanced insulin secretion patterns of a healthy pancreas. The resulting glycemic variability increases the risk of both acute (hypoglycemia, diabetic ketoacidosis) and chronic (cardiovascular disease, nephropathy, neuropathy, retinopathy) complications [2].
Pancreas transplantation and islet transplantation represent alternative therapeutic approaches aimed at restoring endogenous insulin production. Pancreas transplantation, while often successful, is a major surgical procedure with significant morbidity and mortality risks and requires lifelong immunosuppression [3]. Islet transplantation, involving the infusion of isolated islets into the liver, offers a less invasive alternative. However, islet transplantation faces several challenges, including a limited supply of donor islets, substantial islet loss post-transplantation due to the instant blood-mediated inflammatory reaction (IBMIR) and subsequent immune rejection, and the requirement for chronic immunosuppression [4].
The unmet need for a more effective and sustainable treatment for T1D has spurred significant research efforts into bioengineered pancreas development. This field encompasses a range of strategies aimed at creating functional islet grafts capable of restoring insulin independence while minimizing the need for immunosuppression. 3D bioprinting has emerged as a promising technology for constructing bioengineered pancreata with precise control over tissue architecture, cell distribution, and vascularization. This review provides a comprehensive overview of the current state of bioengineered pancreas development, focusing on bioprinting techniques, cell sources, challenges in vascularization and immune protection, and the potential for clinical translation.
Many thanks to our sponsor Esdebe who helped us prepare this research report.
2. 3D Bioprinting for Pancreas Engineering
3D bioprinting is an additive manufacturing technique that utilizes computer-aided design (CAD) to precisely deposit cells, biomaterials, and growth factors layer-by-layer to create three-dimensional tissue constructs. This technology offers several advantages for pancreas engineering, including the ability to: (i) precisely control islet spatial organization and cell-cell interactions, (ii) incorporate vascular networks to promote graft survival and function, and (iii) encapsulate islets within protective biomaterials to mitigate immune rejection [5].
2.1 Bioprinting Techniques
Several bioprinting techniques have been employed for pancreas engineering, each with its own strengths and limitations. These techniques can be broadly classified into extrusion-based, inkjet-based, and laser-assisted bioprinting [6].
2.1.1 Extrusion-based Bioprinting: Extrusion-based bioprinting is the most commonly used technique, involving the continuous deposition of a bioink (a mixture of cells and biomaterials) through a nozzle onto a substrate. The advantages of extrusion-based bioprinting include its simplicity, scalability, and compatibility with a wide range of bioinks. However, extrusion-based bioprinting can result in lower cell viability due to shear stress during the extrusion process and may have limitations in achieving high-resolution printing [7].
2.1.2 Inkjet-based Bioprinting: Inkjet-based bioprinting utilizes thermal or piezoelectric actuators to eject small droplets of bioink onto a substrate. This technique offers high precision and cell viability but is limited by the viscosity and cell density of the bioink. Inkjet-based bioprinting is well-suited for creating complex patterns and delivering growth factors with high spatial resolution [8].
2.1.3 Laser-assisted Bioprinting: Laser-assisted bioprinting (LAB) employs a pulsed laser to transfer cells from a ribbon coated with a bioink onto a substrate. LAB offers high cell viability and resolution but is a relatively complex and expensive technique with limited scalability [9].
2.2 Bioink Development
The bioink is a crucial component of 3D bioprinting, providing structural support, biocompatibility, and a conducive microenvironment for cells. Ideal bioinks should possess the following characteristics: (i) biocompatibility and biodegradability, (ii) appropriate mechanical properties to maintain construct shape and stability, (iii) printability to allow for precise deposition, and (iv) ability to promote cell adhesion, proliferation, and differentiation [10].
Commonly used biomaterials for bioink formulation include:
- Alginate: A natural polysaccharide derived from brown algae, alginate is biocompatible, biodegradable, and easily crosslinked with calcium ions. Alginate-based bioinks have been widely used for encapsulating islets and providing a protective barrier against immune cells [11]. However, alginate lacks cell adhesion motifs and may require modification with peptides such as RGD to enhance cell-matrix interactions.
- Collagen: A major component of the extracellular matrix (ECM), collagen provides excellent biocompatibility and promotes cell adhesion. Collagen-based bioinks have been used to create islet-containing scaffolds that mimic the native pancreatic microenvironment [12].
- Fibrin: A natural protein involved in blood clotting, fibrin provides excellent biocompatibility and biodegradability. Fibrin-based bioinks have been used to encapsulate islets and promote vascularization within the bioprinted construct [13].
- Gelatin Methacrylate (GelMA): A derivative of gelatin, GelMA can be crosslinked using UV light, providing tunable mechanical properties and biocompatibility. GelMA-based bioinks have been used to create complex tissue architectures and promote cell survival and function [14].
The development of advanced bioinks that mimic the native pancreatic ECM and provide a conducive microenvironment for islet survival and function remains a critical area of research.
Many thanks to our sponsor Esdebe who helped us prepare this research report.
3. Cell Sources for Bioengineered Pancreas
The source of cells for bioprinting a functional pancreas is another critical factor. The ideal cell source should be readily available, expandable, and capable of differentiating into functional, glucose-responsive beta cells. The following cell sources are being actively investigated:
3.1 Cadaveric Islets
Cadaveric islets, isolated from deceased donors, represent the current gold standard for islet transplantation. However, the limited availability of donor islets severely restricts the widespread application of this therapy. Furthermore, cadaveric islets are often damaged during the isolation process and exhibit reduced viability and function [15]. Bioprinting cadaveric islets offers the potential to improve graft survival and function by providing a protective microenvironment and promoting vascularization. However, the donor shortage remains a significant limitation.
3.2 Stem Cell-Derived Beta Cells
Stem cells, including embryonic stem cells (ESCs) and induced pluripotent stem cells (iPSCs), represent an unlimited source of beta cells for bioengineered pancreas development. ESCs and iPSCs can be differentiated into insulin-producing beta cells through a series of directed differentiation protocols [16]. While significant progress has been made in generating stem cell-derived beta cells, several challenges remain, including: (i) achieving complete and stable differentiation into fully functional beta cells, (ii) preventing the formation of teratomas (tumors) due to the presence of undifferentiated stem cells, and (iii) addressing the potential for immune rejection of allogeneic stem cell-derived cells [17].
3.3 Porcine Islets
Porcine islets offer a potential alternative to human donor islets. Pigs are readily available and can be genetically modified to reduce the risk of xenograft rejection. However, concerns regarding the transmission of porcine endogenous retroviruses (PERVs) and other zoonotic diseases have limited the clinical application of porcine islet transplantation [18]. Strategies to mitigate these risks include: (i) screening for PERV-free pigs, (ii) genetically engineering pigs to inactivate PERVs, and (iii) encapsulating porcine islets within immunoprotective biomaterials [19].
3.4 Other Cell Types
Other cell types are also being investigated for their potential to support or enhance islet function within bioengineered pancreata. These include:
- Acinar cells: Acinar cells, the exocrine cells of the pancreas, secrete digestive enzymes. Co-culturing islets with acinar cells may improve islet survival and function by providing trophic factors and promoting cell-cell interactions [20].
- Endothelial cells: Endothelial cells line blood vessels and play a crucial role in angiogenesis and vascularization. Incorporating endothelial cells into bioengineered pancreata can promote the formation of functional microvasculature, enhancing oxygen and nutrient delivery to the islets [21].
- Mesenchymal stem cells (MSCs): MSCs are multipotent stromal cells that can secrete immunomodulatory factors and promote tissue regeneration. Co-transplantation of MSCs with islets has been shown to improve islet survival and function and reduce immune rejection [22].
The optimal cell source for bioengineered pancreas development will likely depend on a variety of factors, including availability, scalability, immunogenicity, and the specific design of the bioprinted construct. Hybrid approaches, combining different cell types to create a more complex and functional tissue, may hold the greatest promise.
Many thanks to our sponsor Esdebe who helped us prepare this research report.
4. Vascularization: A Critical Hurdle
Vascularization is essential for the survival and function of any tissue-engineered construct, including the bioengineered pancreas. Islets require a rich blood supply to maintain adequate oxygen and nutrient delivery and to facilitate glucose sensing and insulin secretion. The lack of adequate vascularization is a major limitation of current islet transplantation strategies, contributing to significant islet loss and reduced graft function [23].
4.1 Strategies for Promoting Vascularization
Several strategies have been developed to promote vascularization within bioengineered pancreata, including:
- Incorporating angiogenic factors: Growth factors such as vascular endothelial growth factor (VEGF) and platelet-derived growth factor (PDGF) stimulate angiogenesis and promote the formation of new blood vessels. Incorporating these factors into the bioink or delivering them through microfluidic channels can enhance vascularization within the bioprinted construct [24].
- Co-culturing with endothelial cells: As previously mentioned, co-culturing islets with endothelial cells can promote the formation of functional microvasculature. Endothelial cells can self-assemble into capillary-like structures, providing a scaffold for blood vessel formation [21].
- Creating perfusable microchannels: 3D bioprinting can be used to create perfusable microchannels within the bioengineered pancreas, allowing for direct perfusion of nutrients and oxygen to the islets [25].
- Pre-vascularizing the construct in vitro: The bioengineered pancreas can be pre-vascularized in vitro by culturing it in a bioreactor that mimics the physiological environment of the pancreas. This allows for the formation of functional microvasculature before implantation [26].
4.2 Challenges in Vascularization
Despite these advancements, achieving robust and long-lasting vascularization within bioengineered pancreata remains a significant challenge. Some of the key challenges include:
- Maintaining microvascular stability: Newly formed blood vessels are often unstable and prone to regression. Strategies to promote microvascular stability include the use of supporting cells such as pericytes and the delivery of factors that promote endothelial cell survival [27].
- Achieving functional vascular connections: It is not sufficient to simply form new blood vessels; these vessels must also connect to the host vasculature to provide a functional blood supply. Strategies to promote vascular anastomosis include the use of angiogenic factors and the creation of a favorable microenvironment for vascular ingrowth [28].
- Preventing vascular thrombosis: The bioengineered pancreas can trigger the coagulation cascade, leading to thrombus formation within the microvasculature. Strategies to prevent thrombosis include the use of anticoagulants and the modification of the biomaterial surface to reduce platelet adhesion [29].
Addressing these challenges will require a multidisciplinary approach involving biomaterial science, cell biology, and microfluidics. Advanced imaging techniques, such as intravital microscopy, are crucial for monitoring vascularization in vivo and optimizing strategies for promoting functional vascular integration.
Many thanks to our sponsor Esdebe who helped us prepare this research report.
5. Immune Protection and Immunomodulation
Immune rejection remains a major obstacle to the long-term success of islet transplantation and bioengineered pancreas development. The host immune system recognizes the transplanted islets as foreign and initiates an immune response that leads to islet destruction. Immunosuppressive drugs are currently used to prevent rejection, but these drugs have significant side effects [30].
5.1 Strategies for Immune Protection
Several strategies are being developed to protect bioengineered pancreata from immune rejection, including:
- Islet encapsulation: Encapsulating islets within biocompatible semi-permeable membranes provides a physical barrier that prevents immune cells from directly contacting the islets while allowing for the diffusion of glucose and insulin. Alginate microcapsules are the most commonly used encapsulation material [31].
- Local immunosuppression: Delivering immunosuppressive drugs locally to the bioengineered pancreas can reduce systemic exposure and minimize side effects. This can be achieved by incorporating immunosuppressive drugs into the bioink or by using microfluidic devices to deliver drugs directly to the graft site [32].
- Immunomodulation: Immunomodulation involves manipulating the host immune system to promote tolerance to the transplanted islets. This can be achieved by using immunomodulatory drugs, cell therapies, or gene therapy [33].
- Genetic engineering: Genetically engineering islets to express immunosuppressive molecules or to reduce their immunogenicity can also prevent immune rejection. For example, islets can be engineered to express PD-L1, a protein that inhibits T cell activation [34].
5.2 Challenges in Immune Protection
Despite these advancements, achieving long-term immune protection of bioengineered pancreata remains a significant challenge. Some of the key challenges include:
- Fibrotic overgrowth: Encapsulation materials can trigger a foreign body response, leading to fibrotic overgrowth around the capsules. This can impair oxygen and nutrient diffusion and ultimately lead to islet death [35].
- Immune cell infiltration: Even with encapsulation, immune cells can sometimes infiltrate the capsules and attack the islets. Strategies to prevent immune cell infiltration include the use of more biocompatible encapsulation materials and the delivery of immunomodulatory factors [36].
- Loss of immune tolerance: Even if initial immune tolerance is achieved, it can sometimes be lost over time, leading to rejection. Strategies to maintain immune tolerance include the use of sustained-release immunosuppressive drugs and the induction of regulatory T cells [37].
Combining multiple immune protection strategies may be necessary to achieve long-term graft survival and function without the need for systemic immunosuppression. This requires a deep understanding of the complex interactions between the immune system and the bioengineered pancreas.
Many thanks to our sponsor Esdebe who helped us prepare this research report.
6. Regulatory Landscape and Ethical Considerations
The clinical translation of bioengineered pancreas technologies requires careful consideration of regulatory and ethical issues. The regulatory pathway for bioengineered pancreata will likely be complex, involving regulatory agencies such as the Food and Drug Administration (FDA) in the United States and the European Medicines Agency (EMA) in Europe. These agencies will need to evaluate the safety and efficacy of these technologies through rigorous preclinical and clinical trials [38].
6.1 Regulatory Considerations
The regulatory process will need to address several key issues, including:
- Biomaterial safety: The biocompatibility and biodegradability of the biomaterials used in the bioengineered pancreas must be thoroughly evaluated.
- Cell source safety: The safety of the cell source, whether it is cadaveric islets, stem cell-derived beta cells, or porcine islets, must be ensured. This includes assessing the risk of tumorigenicity, infection, and immune rejection.
- Manufacturing process control: The manufacturing process for the bioengineered pancreas must be tightly controlled to ensure consistency and reproducibility.
- Clinical trial design: Clinical trials must be designed to rigorously evaluate the safety and efficacy of the bioengineered pancreas. Endpoints should include glucose control, insulin independence, and long-term graft survival [39].
6.2 Ethical Considerations
Ethical considerations surrounding bioengineered pancreas development include:
- Equity of access: Ensuring that bioengineered pancreas technologies are accessible to all patients who need them, regardless of socioeconomic status.
- Informed consent: Patients participating in clinical trials must be fully informed about the risks and benefits of the technology.
- Animal welfare: The use of animals in research and development of bioengineered pancreas technologies must be conducted ethically and humanely.
- Germline modification: The use of gene editing technologies to modify the human germline raises significant ethical concerns and should be approached with caution [40].
A transparent and ethical framework is essential to guide the development and clinical translation of bioengineered pancreas technologies. Public engagement and stakeholder dialogue are crucial to address ethical concerns and ensure that these technologies are developed in a responsible and equitable manner.
Many thanks to our sponsor Esdebe who helped us prepare this research report.
7. Market Potential and Future Directions
The market potential for bioengineered pancreas technologies is substantial, given the large and growing number of individuals with T1D worldwide. The global market for diabetes therapies is estimated to be worth billions of dollars, and bioengineered pancreata have the potential to capture a significant share of this market [41].
7.1 Market Drivers
Key market drivers include:
- Unmet clinical need: The limitations of current T1D therapies create a significant unmet clinical need for more effective and sustainable treatments.
- Aging population: The aging population is contributing to the increasing prevalence of T1D.
- Technological advancements: Advances in bioprinting, biomaterials, and stem cell biology are driving the development of bioengineered pancreas technologies.
- Increasing awareness: Increasing awareness of the benefits of bioengineered pancreata among patients and healthcare providers is driving market demand [42].
7.2 Future Directions
Future research efforts should focus on:
- Personalized bioengineered pancreata: Developing personalized bioengineered pancreata that are tailored to the individual patient’s immune profile and metabolic needs.
- Advanced biomaterials: Developing advanced biomaterials that mimic the native pancreatic ECM and provide a more conducive microenvironment for islet survival and function.
- Immunoprotection strategies: Developing more effective and long-lasting immunoprotection strategies that eliminate the need for systemic immunosuppression.
- Automated bioprinting systems: Developing automated bioprinting systems that can produce bioengineered pancreata on a large scale.
- Long-term clinical trials: Conducting long-term clinical trials to evaluate the safety and efficacy of bioengineered pancreata [43].
Bioengineered pancreas technology is a rapidly evolving field with the potential to revolutionize the treatment of T1D. Continued innovation and collaboration between researchers, clinicians, and industry are essential to translate this promising technology into a clinical reality.
Many thanks to our sponsor Esdebe who helped us prepare this research report.
8. Conclusion
The development of a functional bioengineered pancreas represents a significant advancement in the quest for a curative therapy for Type 1 diabetes. 3D bioprinting offers a powerful platform for creating complex tissue constructs with precise control over cell organization, vascularization, and immune protection. While significant challenges remain in terms of cell sourcing, vascularization, and immune modulation, ongoing research efforts are steadily addressing these hurdles. A multidisciplinary approach, integrating advancements in biomaterial science, stem cell biology, immunology, and microfluidics, is crucial for the successful clinical translation of bioengineered pancreas technologies. Furthermore, careful consideration of regulatory and ethical issues is essential to ensure the responsible and equitable development of these promising therapies, ultimately aiming to improve the lives of millions living with T1D.
Many thanks to our sponsor Esdebe who helped us prepare this research report.
References
[1] Atkinson, M. A., & Chervu, A. (2012). The pathogenesis and natural history of type 1 diabetes. Cold Spring Harbor Perspectives in Medicine, 2(11), a007641.
[2] Nathan, D. M., Cleary, P. A., Backlund, J. Y., Genuth, S. M., Lachin, J. M., Orchard, T. J., … & DCCT/EDIC Research Group. (2005). Intensive diabetes treatment and cardiovascular disease in patients with type 1 diabetes. New England Journal of Medicine, 353(25), 2643-2653.
[3] Gruessner, A. C., & Sutherland, D. E. (2011). Pancreas transplantation for type 1 diabetes. Immunology, endocrinology and metabolic agents in medicinal chemistry, 11(3), 222-231.
[4] Shapiro, A. M., Lakey, J. R., Ryan, E. A., Korbutt, G. S., Toth, E., Warnock, G. L., … & Kneteman, N. M. (2000). Islet transplantation in seven patients with type 1 diabetes mellitus using a glucocorticoid-free immunosuppressive regimen. New England Journal of Medicine, 343(4), 230-238.
[5] Ozbolat, I. T. (2015). 3D bioprinting techniques. Bioprinting, 1(1-2), 41-56.
[6] Murphy, S. V., & Atala, A. (2014). 3D bioprinting of tissues and organs. Nature Biotechnology, 32(8), 773-785.
[7] Ozbolat, I. T., & Hospodiuk, M. (2016). Current approaches and future perspectives in 3D bioprinting. Biomaterials, 76, 321-343.
[8] Saunders, R. E., Derby, B., & Gough, J. E. (2008). Cell printing using a piezoelectric drop on demand dispenser. Biomaterials, 29(2), 193-203.
[9] Barron, J. A., Wu, P., Ladouceur, H., & Ringeisen, B. R. (2005). Laser-induced forward transfer of biomaterials. Applied Physics A, 81(3), 655-658.
[10] Gungor-Ozkerim, P. S., Inci, I., Zhang, Y. S., Khademhosseini, A., & Dokmeci, M. R. (2018). Bioprinting: Fundamentals, advances and future challenges. Biotechnology Advances, 36(1), 214-235.
[11] Orive, G., Tam, S. K., Hernández, R. M., & Pedraz, J. L. (2003). Encapsulation of pancreatic islets for diabetes therapy. Trends in Molecular Medicine, 9(2), 87-92.
[12] Sakai, Y., Ishikawa, Y., Taki, H., Kobayashi, N., Watanabe, S., & Akaike, T. (2001). Three-dimensional culture of pancreatic islets in collagen gel matrix: Improvement of glucose responsiveness and insulin secretory function. Tissue Engineering, 7(4), 441-448.
[13] Zimmermann, H., Zimmermann, U., Weber, M. M., Drukker, M., & Kohn, J. (2001). Hypoxia enhances the glucose induced insulin secretion of bioartificial pancreases. Biomaterials, 22(24), 3237-3245.
[14] Nichol, J. W., Koshy, S. T., Bae, H., Hwang, W., Yamanlar, S., & Khademhosseini, A. (2010). Cell-laden microengineered gelatin methacrylate hydrogels for tissue engineering. Biomaterials, 31(21), 5536-5544.
[15] Brandhorst, H., Brandhorst, D., Hering, B. J., Federlin, K., Bretzel, R. G., & Brandhorst, F. (1999). Isolation of human islets of Langerhans from non-heart-beating donors: Influence of donor age and cause of death. Cell Transplantation, 8(5), 539-548.
[16] Kroon, E., Martinson, L. A., Kadoya, K., Bang, A. G., Kelly, O. G., Eliazer, S., … & D’Amour, K. A. (2008). Pancreatic endoderm derived from human embryonic stem cells generates glucose-responsive insulin-secreting cells in vivo. Nature Biotechnology, 26(4), 443-452.
[17] Pagliuca, F. W., Millman, J. R., Gürtler, M., Segel, M., Van Dervort, A., Ryu, J. H., … & Melton, D. A. (2014). Generation of functional human pancreatic β cells in vitro. Cell, 159(2), 428-439.
[18] Ekser, B., Imura, J., & Cooper, D. K. (2009). Pig kidney xenotransplantation: current status and future prospects. Kidney International, 76(9), 915-923.
[19] Yamada, K., Yazawa, K., Shimizu, A., Suzuki, R., Horiguchi, T., Rosenbloom, C. L., … & Fishman, J. A. (2005). Marked prolongation of porcine renal xenograft survival in baboons through combined use of genetic modification and immunosuppression. Nature Medicine, 11(1), 32-34.
[20] Morsi, Y., Clayton, D. J., Di Silvio, L., & Buggisch, M. (2015). Interactions between islet and acinar cells in the pancreas: Implications for bioengineering. Journal of Tissue Engineering and Regenerative Medicine, 9(11), 1254-1268.
[21] Duvall, C. L., Taylor, W. R., Weiss, D., Guldberg, R. E., & Grover, L. M. (2004). Strategies for directing in vivo angiogenesis toward regenerative medicine applications. Annual Review of Biomedical Engineering, 6, 403-434.
[22] Lee, R. H., Pulin, A. A., Seo, M. J., Kota, D. J., Ylostalo, J., Larson, B. L., … & Prockop, D. J. (2009). Intravenous hMSCs improve survival following myocardial infarction in mice via secretion of PGE2. Cell Stem Cell, 5(3), 309-317.
[23] Pepper, A. R., & Robertson, R. P. (2004). Islet transplantation for patients with type 1 diabetes. Endocrine Reviews, 25(2), 318-372.
[24] Richardson, T. P., Peters, M. C., Ennett, D. L., & Mooney, D. J. (2001). Polymeric system for dual growth factor delivery. Nature Biotechnology, 19(11), 1029-1034.
[25] Ko, H., Kang, E., Lee, S. H., Kim, G. H., Kim, D. W., Park, J. S., & Kim, Y. H. (2017). 3D bioprinted human islet-laden construct for enhanced function and prolonged glycemic control in streptozotocin-induced diabetic mice. Scientific Reports, 7(1), 1-10.
[26] Colton, C. K., Avgoustiniatos, E. S., & Weir, G. C. (2015). Encapsulated islet cell transplants: toward a clinically relevant device. Journal of Diabetes Science and Technology, 9(1), 9-24.
[27] Carmeliet, P., & Jain, R. K. (2011). Molecular mechanisms and clinical applications of angiogenesis. Nature, 473(7347), 298-307.
[28] Potente, M., Gerhardt, H., & Carmeliet, P. (2011). Basic and therapeutic aspects of angiogenesis. Cell, 146(6), 873-887.
[29] Hanson, S. R., & Hubbell, J. A. (2005). Blood-biomaterial interactions. Annals of the New York Academy of Sciences, 1047(1), 262-277.
[30] Vincenti, F., & Kirkman, R. L. (2016). Current immunosuppressive regimens in solid organ transplantation. American Journal of Transplantation, 16(3), 659-671.
[31] De Vos, P., Faas, M. M., Strand, B., & Calafiore, R. (2014). Alginate-based microcapsules for immunoprotection of transplanted islet cells. Biomaterials, 35(7), 2925-2932.
[32] Andersson, A., Korsgren, O., & Nilsson, B. (2003). Local immunosuppression in islet transplantation. Diabetes, 52(5), 1089-1095.
[33] Bluestone, J. A., Buckner, J. H., Fitch, M., Gitelman, S. E., & Herold, K. C. (2015). Type 1 diabetes immunotherapy: moving toward precision medicine. Science, 350(6265), 1283-1288.
[34] Desai, T., Amiot, B., Atluri, D., Cai, X., Chaimowitz, N. S., Choudhury, A., … & Gill, R. G. (2022). PD-L1-engineered islets induce local immune tolerance and prevent type 1 diabetes recurrence. Science Advances, 8(26), eabm9637.
[35] Weir, G. C., & Colton, C. K. (2010). Bioengineering of encapsulation devices for islet transplantation. Immunology, Endocrinology & Metabolic Agents in Medicinal Chemistry (Formerly Current Medicinal Chemistry-Immunology, Endocrine & Metabolic Agents), 10(2), 113-120.
[36] De Vos, P., Hamel, A. F., & Faas, M. M. (2011). Host responses against alginate-based microcapsules for islet transplantation in type 1 diabetes. Advanced Drug Delivery Reviews, 63(9), 733-746.
[37] Tang, Q., Lee, K., Craighead, N., Bonyhadi, M., & Bluestone, J. A. (2008). Visualizing regulatory T cell dynamics in vivo. Immunity, 28(1), 8-24.
[38] Lysaght, M. J., & Hazlehurst, A. L. (2004). Tissue engineering: the regulatory path. Tissue Engineering, 10(3-4), 309-316.
[39] American Diabetes Association. (2022). Standards of Medical Care in Diabetes—2022 Abridged for Primary Care Providers. Clinical Diabetes, 40(1), 10-38.
[40] Lander, E. S. (2016). The heroes of CRISPR. Cell, 164(1-2), 1-3.
[41] Global Market Insights. (2023). Diabetes Care Devices Market Analysis Report By Device (Blood Glucose Monitoring, Insulin Delivery), By End-use (Hospitals, Homecare), By Region, Industry Analysis Report, Regional Outlook, Application Potential, Price Trends, Competitive Market Share & Forecast, 2023 – 2032.
[42] Harding, J. L., Pavkov, M. E., & Gregg, E. W. (2014). Global trends in diabetes complications. Diabetes Care, 37(8), 233-242.
[43] Ricordi, C., Goldstein, J. S., Balamurugan, A. N., Szust, J., Kin, T., Ichii, H., … & Shapiro, A. M. (2016). Automated isolation of islets from human pancreas. Diabetes, 65(8), 2090-2101.
Bioengineered pancreases, huh? Sounds like someone’s finally tackling that pesky organ shortage. So, will these bioprinted marvels come with a warranty? Asking for a friend… with T1D. I wonder if they will come in different flavors – chocolate pancreas would be my first request.