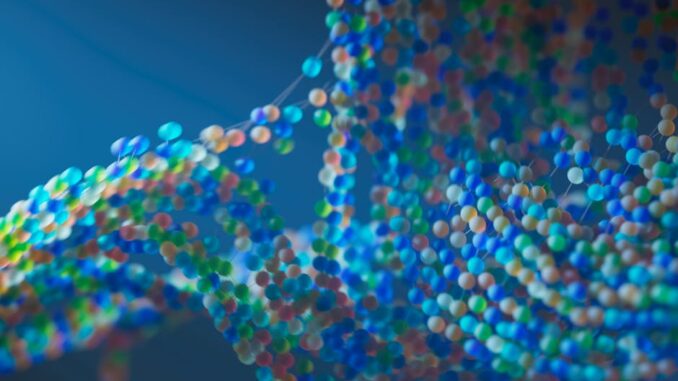
Abstract
Bioluminescence, the production and emission of light by living organisms, has captivated scientists and the public alike for centuries. This phenomenon, driven by intricate biochemical reactions, finds applications across a diverse range of scientific disciplines, from fundamental biological research to advanced medical diagnostics and environmental monitoring. This review delves into the fascinating world of bioluminescence, exploring its underlying mechanisms, the diversity of bioluminescent systems found in nature, and the evolution and engineering of bioluminescent proteins. We discuss recent advancements in bioluminescence imaging (BLI), particularly in the context of live-cell imaging, highlighting the advantages and limitations compared to other imaging modalities. Furthermore, we examine the application of bioluminescence in various fields, including medical diagnostics, drug discovery, synthetic biology, and environmental monitoring. Finally, we address the challenges currently facing bioluminescence research and propose future directions for innovation and expansion of its potential.
Many thanks to our sponsor Esdebe who helped us prepare this research report.
1. Introduction
Bioluminescence, the fascinating ability of living organisms to produce light, represents a remarkable example of biochemical engineering in the natural world [1]. From the ethereal glow of fireflies on a summer night to the deep-sea organisms that illuminate the darkest depths of the ocean, bioluminescence has intrigued humanity for centuries. This phenomenon, based on enzyme-catalyzed chemiluminescent reactions, transcends taxonomic boundaries, occurring in bacteria, fungi, protists, and a wide array of invertebrates and vertebrates [2].
While the initial scientific investigations into bioluminescence were primarily descriptive, focusing on identifying the organisms and basic chemical components involved, modern research has unveiled the intricate molecular mechanisms underlying this process. The discovery of luciferase enzymes and their corresponding substrates (luciferins) paved the way for understanding the biochemistry of light emission. Moreover, advancements in molecular biology, protein engineering, and imaging technologies have revolutionized the field, enabling the development of sophisticated bioluminescent tools for a wide array of applications.
This review aims to provide a comprehensive overview of bioluminescence, covering its history, diversity, mechanisms, and applications. We will explore the different types of bioluminescent systems found in nature, the techniques used to create and modify bioluminescent proteins, and the advantages and disadvantages of bioluminescence compared to other imaging modalities. We will also delve into current and potential applications in various fields, such as medical diagnostics, drug discovery, synthetic biology, and environmental monitoring. Finally, we will discuss the challenges facing bioluminescence research and propose future directions for innovation and expansion of its potential. We will offer our opinion on the relative merits of different approaches and areas of study within the field.
Many thanks to our sponsor Esdebe who helped us prepare this research report.
2. History of Bioluminescence Research
The scientific study of bioluminescence has a rich history spanning several centuries. Early observations and descriptions of bioluminescent organisms date back to ancient civilizations. Aristotle, for instance, described the light emitted by dead fish and fungi in his writings [3]. However, it was not until the 17th century that systematic investigations into the nature of bioluminescence began.
Robert Boyle’s experiments in the late 17th century demonstrated that bioluminescence required air, suggesting an oxidative process [4]. This discovery laid the foundation for understanding the chemical nature of bioluminescence. However, it was not until the late 19th century and early 20th century that significant progress was made in isolating and characterizing the chemical components involved. Raphael Dubois’s work on the bioluminescence of the European click beetle (Pyrophorus plagiophthalamus) led to the identification of two key components: luciferin (the light-emitting substrate) and luciferase (the enzyme catalyzing the reaction) [5].
Dubois’s experiments revealed that luciferin could be extracted from cold water extracts and would react with oxygen catalyzed by luciferase derived from hot water extracts, leading to light emission. E. Newton Harvey further expanded upon Dubois’s work, studying a wide variety of bioluminescent organisms and establishing the generality of the luciferin-luciferase system [6]. Harvey’s monumental work, “Bioluminescence,” published in 1952, remains a cornerstone in the field.
In the latter half of the 20th century, significant advances were made in the purification and characterization of luciferases and luciferins from various organisms. These efforts culminated in the cloning and sequencing of luciferase genes, allowing for the production of recombinant luciferases and the development of bioluminescent reporter assays. The discovery of green fluorescent protein (GFP) in the 1990s further revolutionized the field, providing a powerful tool for visualizing and tracking proteins in living cells [7]. While GFP is technically a fluorescent protein and not a bioluminescent system, its widespread use in live-cell imaging indirectly fueled advancements in bioluminescence research by highlighting the power and utility of in vivo imaging techniques. The integration of GFP and bioluminescence has led to hybrid systems with enhanced capabilities. Furthermore, the rise of synthetic biology has enabled researchers to engineer novel bioluminescent systems with tailored properties, paving the way for new applications in diverse fields.
Many thanks to our sponsor Esdebe who helped us prepare this research report.
3. Diversity of Bioluminescent Systems and Mechanisms
Bioluminescence has evolved independently in diverse lineages of organisms, resulting in a remarkable array of bioluminescent systems. Although the fundamental principle of light emission involves a chemical reaction catalyzed by a luciferase enzyme, the specific luciferins, luciferases, and reaction mechanisms vary considerably across different species [2].
3.1 Bacterial Bioluminescence
Bacterial bioluminescence is perhaps the most well-studied system. It is primarily found in marine bacteria, such as Vibrio fischeri and Photobacterium phosphoreum. The bacterial luciferase is a heterodimeric enzyme that catalyzes the oxidation of reduced flavin mononucleotide (FMNH2) and a long-chain aldehyde (typically tetradecanal) in the presence of oxygen, resulting in the emission of blue-green light [8]. The lux operon, containing the genes encoding the luciferase subunits (luxA and luxB) and other proteins involved in substrate synthesis, regulates bacterial bioluminescence. Quorum sensing, a cell-density-dependent regulatory mechanism, controls light production in many bioluminescent bacteria. This process relies on the production and detection of small signaling molecules (autoinducers) that accumulate as the bacterial population grows, triggering the expression of the lux operon when a critical threshold is reached [9].
3.2 Firefly Bioluminescence
Firefly bioluminescence is arguably the most familiar example of bioluminescence. The firefly luciferase catalyzes the oxidation of luciferin in the presence of ATP, magnesium ions, and oxygen, resulting in the emission of yellow-green light [10]. The precise wavelength of emitted light varies among different firefly species, due to variations in the structure of the luciferase enzyme. Firefly luciferase is widely used as a reporter gene in molecular biology and biotechnology, owing to its high sensitivity, relatively simple assay procedure, and the availability of commercially available reagents.
3.3 Marine Bioluminescence
A vast array of marine organisms, including dinoflagellates, jellyfish, copepods, and fish, exhibit bioluminescence. The bioluminescent systems in these organisms are diverse, with different luciferins, luciferases, and reaction mechanisms. For example, the bioluminescence of dinoflagellates, responsible for the mesmerizing “milky seas,” involves a complex cascade of events initiated by mechanical stimulation. Scintillons, specialized cytoplasmic organelles, contain luciferase, luciferin, and luciferin-binding protein. Mechanical stimulation triggers a pH change in the scintillon, releasing luciferin and initiating the bioluminescent reaction [11]. The bioluminescence of Aequorea victoria, a jellyfish, is mediated by aequorin, a calcium-activated photoprotein. Upon binding calcium ions, aequorin undergoes a conformational change, leading to the oxidation of coelenterazine and the emission of blue light [12]. In some marine organisms, such as copepods, luciferin is derived from their diet, highlighting the importance of food web dynamics in bioluminescence. The diversity and complexity of marine bioluminescent systems underscore the evolutionary adaptations that have shaped this phenomenon in the marine environment.
3.4 Fungal Bioluminescence
Bioluminescence is also observed in a number of fungal species, although it is less common than in marine and bacterial organisms. The luciferin and luciferase involved in fungal bioluminescence have been identified and characterized. Fungal bioluminescence typically emits a green or yellow-green light. The function of bioluminescence in fungi is still not fully understood, but it is hypothesized to play a role in attracting insects for spore dispersal [13].
Many thanks to our sponsor Esdebe who helped us prepare this research report.
4. Engineering and Optimization of Bioluminescent Proteins
The inherent properties of native bioluminescent proteins are not always optimal for specific applications. Therefore, researchers have dedicated considerable effort to engineering and optimizing these proteins to enhance their performance. Various strategies are employed to improve bioluminescence intensity, stability, emission wavelength, and substrate specificity [14].
4.1 Directed Evolution
Directed evolution involves subjecting a gene of interest to random mutagenesis, followed by screening or selection for variants with improved properties. This approach has been successfully used to enhance the activity, stability, and thermostability of luciferases [15]. By iterating through multiple rounds of mutagenesis and selection, researchers can accumulate beneficial mutations that cumulatively improve the desired traits. Directed evolution is particularly useful for optimizing complex properties that are difficult to predict based on structural information alone.
4.2 Rational Design
Rational design involves making targeted modifications to the protein sequence based on structural information and mechanistic understanding. This approach requires detailed knowledge of the protein’s structure and function. By analyzing the active site and surrounding regions, researchers can identify specific amino acid residues that are critical for catalysis, substrate binding, or protein stability. Mutating these residues can lead to significant improvements in enzyme performance. For example, modifying the active site of firefly luciferase can alter its substrate specificity or emission wavelength [16].
4.3 Protein Conjugation and Fusion
Protein conjugation and fusion are used to modify the properties of bioluminescent proteins. For example, conjugating a luciferase to a targeting moiety can direct its localization to a specific cell type or subcellular compartment. Fusing a luciferase to another protein can create a bioluminescent reporter for protein-protein interactions or protein trafficking. Split luciferase assays, in which a luciferase is split into two inactive fragments that can reassemble and become active upon interaction with a target molecule, are also widely used for detecting protein-protein interactions [17].
4.4 Codon Optimization and Expression Systems
Codon optimization involves modifying the DNA sequence of a gene to improve its expression in a particular host organism. Different organisms have different codon preferences, meaning that they utilize certain codons more frequently than others. By optimizing the codon usage of a luciferase gene, researchers can significantly increase its expression level in the chosen host. Furthermore, the selection of an appropriate expression system is crucial for producing high yields of recombinant luciferases. Bacterial, yeast, insect cell, and mammalian cell expression systems are all commonly used, each with its own advantages and disadvantages [18].
Many thanks to our sponsor Esdebe who helped us prepare this research report.
5. Bioluminescence Imaging (BLI) and Live-Cell Applications
Bioluminescence imaging (BLI) is a powerful non-invasive technique for visualizing biological processes in living organisms [19]. In BLI, a bioluminescent reporter gene, such as luciferase, is introduced into cells or animals, and the emitted light is detected using a sensitive camera. BLI offers several advantages over other imaging modalities, including high sensitivity, low background signal, and the ability to perform longitudinal studies. These advantages make it particularly well-suited for live-cell imaging applications.
5.1 Advantages of BLI
- High Sensitivity: BLI is exceptionally sensitive, allowing the detection of even small numbers of bioluminescent cells or molecules. This sensitivity stems from the fact that bioluminescence generates photons directly, without requiring external excitation light, thus minimizing background noise.
- Low Background Signal: The absence of external excitation light also results in a very low background signal, enhancing the signal-to-noise ratio. This is a significant advantage over fluorescence imaging, where autofluorescence from endogenous molecules can interfere with the signal of interest.
- Non-Invasive: BLI is a non-invasive technique, as it does not require the administration of harmful substances or exposure to high-energy radiation. This makes it suitable for longitudinal studies, where the same subject can be imaged repeatedly over time.
- Real-Time Monitoring: BLI allows for real-time monitoring of biological processes, providing dynamic information about cellular activity and molecular interactions.
5.2 Limitations of BLI
- Light Attenuation: Light emitted from bioluminescent sources within tissues can be attenuated by absorption and scattering, reducing the signal intensity and spatial resolution. This is a significant limitation, especially for deep-tissue imaging. Various strategies, such as using luciferases with red-shifted emission spectra, are being developed to minimize light attenuation [20].
- Spatial Resolution: The spatial resolution of BLI is limited by light scattering and the sensitivity of the camera. It is generally lower than that of other imaging modalities, such as microscopy or magnetic resonance imaging (MRI). However, advancements in camera technology and image reconstruction algorithms are improving the spatial resolution of BLI.
- Substrate Delivery: Efficient delivery of the luciferase substrate (luciferin) to the target cells or tissues is crucial for optimal bioluminescence signal. Poor substrate delivery can limit the sensitivity and accuracy of BLI. Various strategies, such as using injectable luciferin formulations or genetically encoding luciferin biosynthesis pathways, are being explored to improve substrate delivery [21].
- Metabolic Perturbation: The bioluminescent reaction requires substrates and cofactors (e.g., ATP, oxygen) that may be limited in some cellular environments. The consumption of these molecules during light emission can potentially perturb cellular metabolism and affect the results of the experiment. Careful experimental design and appropriate controls are necessary to minimize these effects.
5.3 Applications in Live-Cell Imaging
BLI has a wide range of applications in live-cell imaging, including:
- Gene Expression Studies: Luciferase reporter assays are widely used to study gene expression in living cells. By placing the luciferase gene under the control of a specific promoter, researchers can monitor the activity of that promoter in real-time [22].
- Protein-Protein Interactions: Split luciferase assays are used to detect protein-protein interactions in living cells. When two proteins of interest interact, the two luciferase fragments reassemble, resulting in bioluminescence signal [17].
- Cell Tracking: BLI can be used to track the movement and fate of cells in living organisms. For example, bioluminescently labeled cancer cells can be tracked to monitor tumor growth and metastasis [23].
- Drug Discovery: BLI is used in drug discovery to screen for compounds that affect cellular signaling pathways or protein activity. High-throughput BLI assays can be used to identify potential drug candidates [24].
- Monitoring Cellular Metabolism: Genetically encoded bioluminescent sensors are being developed to monitor cellular metabolites, such as ATP, calcium, and reactive oxygen species. These sensors provide real-time information about cellular metabolism and can be used to study a variety of physiological and pathological processes [25].
Many thanks to our sponsor Esdebe who helped us prepare this research report.
6. Applications in Diverse Fields
Beyond live-cell imaging, bioluminescence has found applications in a diverse range of fields, including medical diagnostics, drug discovery, environmental monitoring, and synthetic biology.
6.1 Medical Diagnostics
Bioluminescence is used in medical diagnostics for a variety of purposes, including:
- Infectious Disease Detection: Bioluminescent bacteria or bacteriophages can be used to detect pathogenic microorganisms in clinical samples. The presence of the pathogen is indicated by bioluminescence signal [26].
- Cancer Detection and Imaging: BLI is used to image tumors in preclinical models and is being explored for cancer detection in humans. Bioluminescent probes can be designed to target specific tumor markers, enhancing the sensitivity and specificity of the imaging [23].
- Monitoring Immune Responses: BLI can be used to monitor immune cell activity in vivo. Bioluminescently labeled immune cells can be tracked to assess their trafficking and function in response to infection or inflammation [27].
6.2 Drug Discovery
Bioluminescence is used in drug discovery for a variety of purposes, including:
- High-Throughput Screening: BLI assays are used to screen large libraries of compounds for their effects on cellular signaling pathways, protein activity, or gene expression [24].
- Drug Metabolism and Pharmacokinetics: BLI can be used to monitor the metabolism and pharmacokinetics of drugs in vivo. Bioluminescently labeled drugs can be tracked to assess their distribution, metabolism, and excretion [28].
- Drug Efficacy Studies: BLI is used to assess the efficacy of drugs in preclinical models. For example, BLI can be used to monitor tumor growth in response to chemotherapy [23].
6.3 Environmental Monitoring
Bioluminescence is used in environmental monitoring for a variety of purposes, including:
- Water Quality Assessment: Bioluminescent bacteria can be used to assess water quality. The presence of pollutants in the water can inhibit bacterial bioluminescence, providing a sensitive indicator of water contamination [29].
- Detection of Toxic Compounds: Bioluminescent biosensors can be designed to detect specific toxic compounds in the environment. These biosensors typically consist of a bioluminescent bacterium or enzyme that is sensitive to the target compound [30].
6.4 Synthetic Biology
Synthetic biology aims to design and construct new biological systems with novel functions. Bioluminescence is a powerful tool for synthetic biology, as it provides a convenient and sensitive way to monitor the activity of engineered biological circuits [31]. For example, bioluminescent reporters can be used to monitor the expression of engineered genes or the activity of synthetic metabolic pathways. Bioluminescent communication systems can be engineered to allow cells to communicate with each other using light signals [32].
Many thanks to our sponsor Esdebe who helped us prepare this research report.
7. Challenges and Future Directions
While bioluminescence has emerged as a powerful tool with diverse applications, several challenges remain. Addressing these challenges will be critical for further advancing the field and expanding its potential.
7.1 Improving Light Output and Tissue Penetration
One major challenge is to improve the light output of bioluminescent systems and to enhance the penetration of light through tissues. This can be achieved through several strategies, including:
- Developing More Efficient Luciferases: Research efforts are focused on engineering luciferases with higher catalytic activity and quantum yields. This can be achieved through directed evolution, rational design, or a combination of both [15, 16].
- Using Red-Shifted Luciferases: Red light penetrates tissues more effectively than blue or green light. Therefore, developing luciferases with red-shifted emission spectra can improve the sensitivity of BLI in deep tissues [20].
- Optimizing Substrate Delivery: Efficient delivery of luciferin to the target cells or tissues is crucial for optimal bioluminescence signal. Various strategies, such as using injectable luciferin formulations or genetically encoding luciferin biosynthesis pathways, are being explored to improve substrate delivery [21].
- Developing New Luciferins: Novel luciferin analogs with improved properties, such as higher stability, bioavailability, and light output, are being synthesized and evaluated [33].
7.2 Expanding the Palette of Bioluminescent Colors
Expanding the palette of bioluminescent colors would enable the simultaneous monitoring of multiple biological processes in the same cell or organism. This can be achieved through several strategies, including:
- Engineering Luciferases with Different Emission Spectra: By modifying the active site of luciferases, researchers can alter their emission spectra. This can be achieved through directed evolution or rational design [16].
- Developing Resonance Energy Transfer (BRET) Systems: BRET involves the transfer of energy from a bioluminescent donor to a fluorescent acceptor, resulting in a shift in the emission spectrum. BRET systems can be used to create a variety of bioluminescent colors [34].
7.3 Developing Genetically Encoded Bioluminescent Sensors
Genetically encoded bioluminescent sensors provide a powerful way to monitor cellular processes in real-time. Future research efforts will focus on developing new sensors for a wider range of analytes, including metabolites, ions, and signaling molecules [25]. These sensors will enable a deeper understanding of cellular function and will have applications in drug discovery, diagnostics, and synthetic biology.
7.4 Improving Spatial Resolution of BLI
Improving the spatial resolution of BLI is crucial for visualizing fine details of biological processes. This can be achieved through several strategies, including:
- Developing High-Resolution Cameras: Advancements in camera technology are leading to the development of cameras with higher sensitivity and spatial resolution.
- Using Image Reconstruction Algorithms: Image reconstruction algorithms can be used to improve the spatial resolution of BLI images by correcting for light scattering and absorption [35].
- Developing Bioluminescent Microscopes: Bioluminescent microscopes combine the advantages of bioluminescence and microscopy, enabling high-resolution imaging of bioluminescent processes in cells and tissues.
7.5 Increasing Adoption and Standardization
To increase the adoption and impact of bioluminescence technologies, standardization of methods and reagents is essential. This includes the development of validated protocols for BLI, standardized luciferase assays, and well-characterized bioluminescent proteins. Furthermore, promoting collaboration and data sharing among researchers will accelerate the development and application of bioluminescence technologies. The inherent interdisciplinary nature of the field, bridging biology, chemistry, and engineering, requires a collaborative environment to foster innovation and address the complex challenges ahead.
Many thanks to our sponsor Esdebe who helped us prepare this research report.
8. Conclusion
Bioluminescence, a captivating phenomenon with a rich history, has evolved into a powerful and versatile tool for scientific exploration. From unraveling fundamental biological mechanisms to enabling cutting-edge applications in medical diagnostics, drug discovery, and environmental monitoring, bioluminescence continues to shape diverse fields. The ongoing advancements in protein engineering, imaging technologies, and synthetic biology are further expanding the potential of bioluminescence, paving the way for new discoveries and innovations. While challenges remain, the future of bioluminescence research is bright, promising exciting breakthroughs that will deepen our understanding of life and improve human health. The field’s continued growth hinges on addressing current limitations such as improving light output and tissue penetration, as well as promoting standardization and collaboration among researchers. The promise of real-time, non-invasive imaging and sensing continues to drive innovation, solidifying bioluminescence as a cornerstone of modern biological research and technological advancement.
Many thanks to our sponsor Esdebe who helped us prepare this research report.
References
[1] Wilson, T., & Hastings, J. W. (1998). Bioluminescence. Annual review of cell and developmental biology, 14(1), 197-230.
[2] Haddock, S. H. D., Moline, M. A., & Case, J. F. (2010). Bioluminescence in the sea. Annual review of marine science, 2, 443-493.
[3] Aristotle. (350 BC). De Anima.
[4] Boyle, R. (1667). Experiments concerning the relation between light and air. Philosophical Transactions of the Royal Society of London, 2, 581-600.
[5] Dubois, R. (1887). Fonction photogénique chez les pholades. Comptes rendus de la Société de biologie, 9, 564-566.
[6] Harvey, E. N. (1952). Bioluminescence. Academic Press.
[7] Chalfie, M., Tu, Y., Euskirchen, G., Ward, W. W., & Prasher, D. C. (1994). Green fluorescent protein as a marker for gene expression. Science, 263(5148), 802-805.
[8] Meighen, E. A. (1991). Molecular biology of bacterial bioluminescence. Microbiological reviews, 55(1), 123-142.
[9] Nealson, K. H., Platt, T., & Hastings, J. W. (1970). Cellular control of the synthesis and activity of the bacterial luminescent system. Journal of bacteriology, 104(1), 313-322.
[10] Branchini, B. R., Ablamsky, D. M., Murtiashaw, M. H., Uzasci, L., Southworth, T. L., & Kricka, L. J. (2007). Luciferase substrates, emerging chemiluminescent labels, and bioluminescent applications. Analytical biochemistry, 361(1), 1-19.
[11] Hastings, J. W. (1983). Biological diversity, chemical mechanisms, and the evolutionary origins of bioluminescent systems. Journal of Molecular Evolution, 19(5), 309-321.
[12] Shimomura, O. (1995). A short story of aequorin. Biological Bulletin, 189(1), 1-5.
[13] Desjardin, D. E., & Oliveira, A. G. (2007). Luminescent Mycena: taxonomic perspectives. Mycological Research, 111(6), 585-602.
[14] De A, A., Loening, A. M., Gambhir, S. S., & Rao, J. (2009). Improving bioluminescence resonance energy transfer (BRET) by directed evolution. ACS chemical biology, 4(11), 929-933.
[15] White, P. J., Hall, M. P., Fraga, H., Daunert, S., & Deo, S. K. (2011). Directed evolution of luciferase for improved bioluminescence resonance energy transfer. ACS chemical biology, 6(5), 491-500.
[16] Nakajima, Y., Yamazaki, T., Nishizaki, S., Ikeda, M., Kuramitsu, S., & Nakatani, H. (2005). Tuning the color of firefly bioluminescence by single amino acid substitutions. FEBS letters, 579(11), 2737-2740.
[17] Remy, I., Campbell-Valois, F. X., & Michnick, S. W. (2007). Detection of protein-protein interactions using protein-fragment complementation assay. Nature protocols, 2(7), 1429-1445.
[18] Hussain, M. A., & Daunert, S. (2015). Expression of luciferase and its applications in biosensing. Sensors (Basel, Switzerland), 15(8), 19626-19654.
[19] Rice, B. W., Cable, M. D., & Nelson, M. B. (2001). In vivo bioluminescence imaging. Nature protocols, 6(6), 721-734.
[20] Smith, A. M., Mancini, M. C., & Nie, S. (2009). Second near-infrared window (NIR-II) imaging for biomedical applications. Accounts of chemical research, 42(8), 1094-1103.
[21] Knox, A. J., Bolcaen, J., Van Holle, S., Everaert, C., & De Backer, P. (2015). Bioluminescence substrate delivery: state of the art and future perspectives. Journal of biomedical optics, 20(8), 080901.
[22] Bronstein, I., Fortin, J., Voyta, J. C., Juo, R. R., Laughlin, M. M., & Olesen, C. E. D. (1994). Chemiluminescent and bioluminescent reporter gene assays. Analytical biochemistry, 219(2), 169-181.
[23] Weissleder, R. (2001). Scaling down imaging: molecular mapping of cancer in mice. Nature Reviews Cancer, 2(1), 11-18.
[24] Thorne, N., Inglese, J., & Auld, D. S. (2010). Illuminating insights into firefly luciferase and bioluminescent assays for drug discovery. Assay and drug development technologies, 8(6), 682-699.
[25] Tantama, M., Culbertson, S. D., & Yellen, G. (2013). Quantitative ratiometric imaging of glutamate with genetically encoded sensors. Nature chemical biology, 9(11), 724-732.
[26] Ulitzur, S., & Kuhn, J. (1988). Light-emitting bacteria as analytical tools. Microbiological sciences, 5(3), 80-83.
[27] Burns, J. S., Verhoog, S., Smith, B. A., Waldmann, T. A., & Adelman, D. C. (2007). Noninvasive in vivo imaging of murine T cell trafficking using luciferase-expressing T cells. Blood, 110(1), 345-354.
[28] Pysz, M. A., Foygel, K., Chaudhari, A., Kuruppu, D., Patel, P., & Willmann, J. K. (2010). Bioluminescence imaging of drug delivery and its use in personalized medicine. Advanced drug delivery reviews, 62(12), 1225-1237.
[29] Steinberg, S. M., Poziomek, E. J., Engelmann, W. H., & Rogers, K. R. (1995). A simple bioluminescence assay for toxicity screening. Chemosphere, 30(11), 2155-2164.
[30] D’Orazio, P. (2005). Biosensors in clinical chemistry. Clinica Chimica Acta, 360(1-2), 1-14.
[31] Khalil, A. S., & Collins, J. J. (2010). Synthetic biology: applications come of age. Nature Reviews Genetics, 11(5), 367-379.
[32] Danino, T., Mondragón-Palomino, O., Tsimring, L. S., & Hasty, J. (2010). Synthetic quorum sensing circuit to control consortial biofilm formation. Nature, 463(7279), 326-330.
[33] Gurzadyan, G. G., Gorokhova, E. S., Vasilyev, Y. V., Karamyan, V. T., Lukyanov, S. A., & Zaraisky, A. G. (2010). Enhanced photostability of red fluorescent proteins and their application for two-photon in vivo imaging. PLoS One, 5(11), e14732.
[34] Pfleger, K. D. G., & Eidne, K. A. (2006). Bioluminescence resonance energy transfer (BRET) for the real-time detection of protein-protein interactions. Nature protocols, 1(7), 356-363.
[35] Denoël, T., Vanhove, C., Pourcelot, L., & Desreux, J. F. (2004). Improved image reconstruction in bioluminescence tomography using a priori anatomical information. Physics in medicine and biology, 49(17), 3517.
Given the diversity of bioluminescent systems, are there unifying principles governing the evolution of luciferases and luciferins across disparate species? Could identifying such principles accelerate the design of novel bioluminescent tools with tailored properties?
That’s a great question! Identifying unifying evolutionary principles could definitely revolutionize how we design new bioluminescent tools. Comparative genomics and structural analysis might reveal conserved domains or motifs crucial for function. Understanding these commonalities could allow us to engineer luciferases with predictable and tailored properties. Thanks for sparking this discussion!
Editor: MedTechNews.Uk
Thank you to our Sponsor Esdebe
The review highlights the potential of red-shifted luciferases for improved tissue penetration. Has anyone explored combining these with nanoparticles for targeted delivery, potentially enhancing both signal strength and resolution in deep-tissue bioluminescence imaging?
That’s a really insightful question! I haven’t seen studies combining red-shifted luciferases with nanoparticles directly, but that approach holds immense promise. Enhanced signal strength & resolution could really open doors for deep-tissue imaging. I wonder if anyone in the group has relevant experience or publications to share?
Editor: MedTechNews.Uk
Thank you to our Sponsor Esdebe