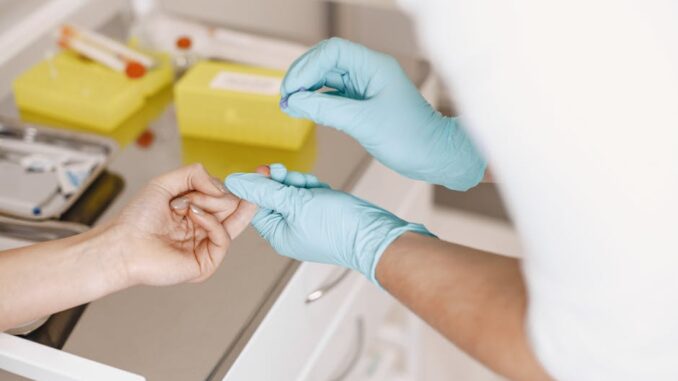
Abstract
Circulating tumor DNA (ctDNA), a fragmented form of DNA released from tumor cells into the bloodstream, has emerged as a powerful tool for cancer management. This ‘liquid biopsy’ offers a non-invasive means to capture a real-time molecular snapshot of the tumor, overcoming limitations associated with traditional tissue biopsies. This review delves into the complex biology of ctDNA shedding, the diverse methodologies employed for its extraction and analysis, and its burgeoning clinical applications, with a particular emphasis on pediatric oncology. We discuss the mechanisms underlying ctDNA release, factors influencing its detectability, and the technical challenges associated with its isolation and characterization, especially given its often low abundance. Furthermore, we explore the current and potential clinical utility of ctDNA in pediatric cancers, including early cancer detection (though still in its infancy), minimal residual disease (MRD) monitoring, treatment response assessment, identification of resistance mechanisms, and personalized therapy selection. Finally, we critically evaluate the existing literature, highlight the ongoing challenges and future directions, and offer insights into the potential of ctDNA to transform cancer care, particularly in the context of pediatric malignancies, where minimizing invasive procedures is paramount.
Many thanks to our sponsor Esdebe who helped us prepare this research report.
1. Introduction
The paradigm shift from relying solely on tissue biopsies to embracing liquid biopsies has revolutionized cancer diagnostics and monitoring. Among the various components of liquid biopsies, ctDNA stands out as a particularly promising biomarker. The presence of tumor-specific genetic alterations within ctDNA provides valuable information about the tumor’s molecular profile, tumor burden, and dynamic changes in response to therapy. This is especially significant in pediatric oncology, where obtaining tissue biopsies can be ethically challenging and clinically difficult due to the invasive nature of the procedure and the limited availability of tissue from young patients. Furthermore, childhood cancers are often characterized by unique genomic landscapes and rapid disease progression, making real-time monitoring through ctDNA particularly crucial. The information from ctDNA can be applied in early detection, treatment monitoring, and identifying resistance mechanisms. Despite significant advances, challenges remain in optimizing ctDNA detection and analysis, particularly in pediatric cancers, where tumor-specific DNA is often present at very low concentrations. In this review, we provide a comprehensive overview of ctDNA biology, extraction methods, analytical techniques, and its clinical utility in pediatric oncology, with a focus on current challenges and future directions.
Many thanks to our sponsor Esdebe who helped us prepare this research report.
2. ctDNA Shedding: Mechanisms and Factors Influencing Detection
The release of ctDNA into the circulation is a complex process influenced by several factors, including tumor biology, the tumor microenvironment, and host-related variables. The primary mechanism of ctDNA shedding is thought to be apoptosis and necrosis of tumor cells. During these processes, DNA is fragmented and released into the extracellular space, ultimately entering the bloodstream. However, other mechanisms such as active secretion of DNA-containing exosomes or microvesicles may also contribute to ctDNA release [1].
The amount of ctDNA in circulation can vary significantly depending on several factors. First, tumor burden is a major determinant. Larger tumors generally release more ctDNA compared to smaller tumors. However, other factors such as tumor location, vascularity, and growth rate can also influence ctDNA levels. Tumors with high cell turnover rates, such as those undergoing rapid proliferation or significant cell death, may release more ctDNA. The degree of tumor vascularization also plays a role, as increased blood supply to the tumor facilitates the entry of ctDNA into the circulation. The specific cancer type and its stage also play a role, with some cancer types, such as leukemia, have been shown to contain more ctDNA than other solid tumors.
Host-related factors, such as immune response and clearance mechanisms, can also impact ctDNA detection. The immune system can recognize and eliminate ctDNA, reducing its levels in circulation. Clearance by the liver and kidneys also contributes to ctDNA removal. Furthermore, factors such as inflammation and infection can influence the overall levels of cell-free DNA (cfDNA) in circulation, which can dilute the ctDNA signal and make its detection more challenging [2].
In pediatric cancers, ctDNA shedding and detection can be particularly challenging due to several factors. Pediatric tumors are often characterized by unique genomic landscapes and lower mutational burdens compared to adult cancers. This means that the fraction of ctDNA carrying tumor-specific mutations may be lower, making it more difficult to distinguish from background cfDNA. Furthermore, pediatric patients often have smaller body sizes and lower blood volumes, which can limit the amount of sample available for ctDNA analysis. Given the influence of these factors, careful consideration of pre-analytical variables, such as blood collection protocols and cfDNA extraction methods, is essential for maximizing ctDNA detection rates in pediatric oncology. Techniques to enrich for tumor-specific DNA can also be vital for increasing the sensitivity of ctDNA analysis in pediatric cancers [3].
Many thanks to our sponsor Esdebe who helped us prepare this research report.
3. ctDNA Extraction and Analysis: Methods and Technical Challenges
3.1. ctDNA Extraction Methods
ctDNA extraction is a critical step in the liquid biopsy workflow. The goal is to efficiently isolate cfDNA from plasma or serum samples, while minimizing contamination and maximizing DNA recovery. Several methods are available for ctDNA extraction, each with its own advantages and limitations. Common techniques include:
-
Silica-based extraction: This is one of the most widely used methods for cfDNA extraction. It relies on the affinity of DNA for silica in the presence of chaotropic salts. The cfDNA is bound to a silica membrane or beads, washed to remove contaminants, and then eluted with a low-salt buffer. Silica-based methods are generally robust, cost-effective, and readily adaptable to automated platforms. Kits are available such as QIAamp Circulating Nucleic Acid Kit (Qiagen). However, they can suffer from lower recovery rates compared to other methods, particularly for low-abundance cfDNA fragments. The presence of inhibitors from the plasma can also affect the PCR later in the process. Modification of the silica surface can help to overcome these issues [4].
-
Magnetic bead-based extraction: This method utilizes magnetic beads coated with a DNA-binding reagent. The cfDNA binds to the beads, which are then separated from the sample using a magnetic field. After washing, the cfDNA is eluted from the beads. Magnetic bead-based methods offer several advantages, including high throughput, automation capability, and improved recovery rates compared to silica-based methods. However, they can be more expensive. Kits include MagMAX Cell-Free DNA Isolation Kit (ThermoFisher). The size selection properties of the beads can also be tuned to improve sensitivity [5].
-
Organic extraction: Traditional organic extraction methods, such as phenol-chloroform extraction, are rarely used for cfDNA isolation due to their toxicity and labor-intensive nature. However, they can provide high DNA yields and purity. Newer modifications have mitigated some of the concerns with the classical methods, such as using phase separation to purify DNA.
-
Size-selection methods: cfDNA is typically fragmented into smaller sizes (approximately 150-200 base pairs). Size-selection methods aim to enrich for these smaller fragments, thereby increasing the concentration of ctDNA relative to larger fragments of non-tumor origin. Size-selection can be achieved through various techniques, including gel electrophoresis, microfluidic devices, or magnetic bead-based methods.
-
Hybrid Capture: This approach uses biotinylated probes that are complementary to regions of the genome that contain mutations that are specific to the tumor. This approach can isolate both targeted single genes, or the whole exome. Kits include IDT xGen Prism DNA Library Prep Kit.
The choice of extraction method depends on several factors, including the required DNA yield and purity, the downstream analytical techniques, the sample volume, and the availability of resources. For ctDNA analysis in pediatric oncology, where tumor-specific DNA is often present at very low concentrations, optimizing the extraction protocol is crucial. This may involve using methods with high recovery rates and incorporating size-selection steps to enrich for ctDNA fragments [6].
3.2. ctDNA Analysis Techniques
Once cfDNA has been extracted, a variety of analytical techniques can be used to detect and characterize ctDNA. These techniques can be broadly classified into two categories: targeted and untargeted approaches.
-
Targeted approaches: Targeted approaches focus on detecting specific mutations or alterations known to be present in the tumor. These methods are highly sensitive and can detect ctDNA even when it is present at very low concentrations. Commonly used targeted techniques include:
- Digital PCR (dPCR): dPCR is a highly sensitive and quantitative technique that allows for the absolute quantification of target DNA sequences. The sample is partitioned into thousands of individual reaction chambers, each containing either zero or one copy of the target DNA molecule. The number of positive reactions is then counted to determine the absolute quantity of the target sequence. dPCR is particularly useful for detecting low-frequency mutations and monitoring minimal residual disease (MRD). However, it is limited to analyzing a small number of targets per reaction. The sensitivity of dPCR is extremely high, and can identify one mutation in hundreds of thousands of fragments.
- Amplicon sequencing: Amplicon sequencing involves amplifying specific regions of the genome containing known mutations and then sequencing the amplified products. This allows for the detection of multiple mutations simultaneously. However, the sensitivity of amplicon sequencing is lower compared to dPCR. Sophisticated bioinformatic tools can be used to identify mutations.
- Targeted next-generation sequencing (NGS): Targeted NGS panels are designed to interrogate a predefined set of genes or genomic regions known to be frequently mutated in cancer. This approach offers higher throughput and can detect a broader range of mutations compared to dPCR and amplicon sequencing. However, the cost per sample is typically higher, and the sensitivity may be lower for detecting very low-frequency mutations.
-
Untargeted approaches: Untargeted approaches aim to identify novel mutations or alterations without prior knowledge of the tumor’s genomic profile. These methods are particularly useful for identifying resistance mechanisms or tracking tumor evolution. Commonly used untargeted techniques include:
- Whole-exome sequencing (WES): WES involves sequencing the entire protein-coding region of the genome. This allows for the detection of all mutations present in the exome, including both known and novel mutations. WES is a powerful tool for identifying resistance mechanisms and tracking tumor evolution. However, it is expensive and requires significant computational resources. Furthermore, the analysis of WES data can be complex, and it may be challenging to distinguish between driver mutations and passenger mutations.
- Whole-genome sequencing (WGS): WGS involves sequencing the entire genome, including both coding and non-coding regions. This provides the most comprehensive view of the tumor’s genomic landscape. WGS can identify structural variations, copy number alterations, and mutations in non-coding regions that may play a role in cancer development and progression. However, WGS is even more expensive and computationally intensive than WES. The interpretation of WGS data is also challenging, and it may be difficult to distinguish between functionally relevant alterations and background noise.
3.3. Technical Challenges in ctDNA Analysis
Despite the significant advances in ctDNA extraction and analysis, several technical challenges remain, particularly in pediatric oncology. These challenges include:
- Low ctDNA concentration: As mentioned earlier, ctDNA is often present at very low concentrations in pediatric patients, especially in early-stage disease or after treatment. This makes it difficult to detect ctDNA and can limit the sensitivity of ctDNA-based assays. Techniques to enrich for ctDNA fragments or to amplify the ctDNA signal are essential for overcoming this challenge.
- High background cfDNA: The majority of cfDNA in circulation is derived from non-tumor cells. This high background cfDNA can dilute the ctDNA signal and make it more difficult to distinguish from background noise. Methods to reduce the background cfDNA or to selectively enrich for ctDNA are needed to improve the sensitivity of ctDNA analysis. Techniques such as using unique molecular identifiers (UMIs) to reduce PCR errors can help differentiate true ctDNA from artifacts [7].
- Tumor heterogeneity: Tumors are often heterogeneous, meaning that different regions of the tumor may have different genetic profiles. This can lead to variability in ctDNA shedding and can make it difficult to accurately represent the entire tumor using ctDNA analysis. Serial ctDNA monitoring can help to capture the dynamic changes in the tumor’s genomic profile over time. This is especially relevant in cases where tumors develop resistance mechanisms.
- Lack of standardized protocols: The lack of standardized protocols for ctDNA extraction, analysis, and data interpretation can lead to variability in results between different laboratories. This can make it difficult to compare results across different studies and can limit the clinical utility of ctDNA testing. Efforts are underway to develop standardized protocols and quality control measures for ctDNA analysis.
- Bioinformatic challenges: The analysis of ctDNA data can be complex and requires specialized bioinformatic tools and expertise. Distinguishing between true mutations and sequencing errors can be challenging, particularly when ctDNA is present at very low concentrations. Developing robust bioinformatic pipelines and using appropriate statistical methods are essential for accurate ctDNA analysis.
Many thanks to our sponsor Esdebe who helped us prepare this research report.
4. Clinical Applications of ctDNA Testing in Pediatric Oncology
ctDNA testing holds great promise for improving the management of pediatric cancers. Its non-invasive nature and ability to provide real-time information about the tumor make it an attractive tool for various clinical applications.
4.1. Early Cancer Detection
While ctDNA analysis is not currently used for routine cancer screening in pediatric populations, it has the potential to play a role in early cancer detection, particularly in high-risk individuals. For example, children with inherited cancer predisposition syndromes, such as Li-Fraumeni syndrome or retinoblastoma, may benefit from ctDNA monitoring to detect cancer at an earlier stage. Challenges remain in achieving sufficient sensitivity and specificity for early cancer detection, especially given the low ctDNA concentrations in early-stage disease. Furthermore, the cost and complexity of ctDNA testing need to be considered when evaluating its potential for early cancer detection [8].
4.2. Minimal Residual Disease (MRD) Monitoring
MRD monitoring is one of the most promising applications of ctDNA testing in pediatric oncology. MRD refers to the presence of residual cancer cells after treatment, which can lead to relapse. Traditional methods for MRD detection, such as bone marrow aspirates and flow cytometry, are invasive and may not be sensitive enough to detect low levels of residual disease. ctDNA testing offers a non-invasive and highly sensitive alternative for MRD monitoring. By detecting tumor-specific mutations in ctDNA, it is possible to identify patients who are at high risk of relapse and to tailor treatment accordingly. Studies have shown that ctDNA-based MRD monitoring can predict relapse in pediatric acute lymphoblastic leukemia (ALL) and neuroblastoma [9].
4.3. Treatment Response Assessment
ctDNA analysis can be used to monitor treatment response in real-time. Changes in ctDNA levels during treatment can provide valuable information about the effectiveness of the therapy. For example, a decrease in ctDNA levels may indicate that the treatment is working, while an increase in ctDNA levels may suggest that the tumor is resistant to the therapy. ctDNA monitoring can also help to identify patients who are not responding to treatment early on, allowing for timely adjustments to the treatment plan. This is particularly important in pediatric oncology, where children may experience significant side effects from ineffective therapies. Longitudinal monitoring of ctDNA during treatment can inform decisions about treatment duration, dose adjustments, or changes in treatment strategy [10].
4.4. Identification of Resistance Mechanisms
ctDNA analysis can be used to identify resistance mechanisms that emerge during treatment. By analyzing ctDNA samples collected at different time points during treatment, it is possible to track the evolution of the tumor’s genomic profile and to identify mutations that confer resistance to the therapy. This information can be used to select alternative therapies that target the resistance mechanisms. For example, if ctDNA analysis reveals the emergence of a mutation in a specific gene, a targeted therapy that inhibits that gene may be effective. ctDNA analysis can also help to identify patients who are likely to develop resistance in the future, allowing for proactive interventions to prevent resistance from occurring [11].
4.5. Personalized Therapy Selection
ctDNA analysis can be used to guide personalized therapy selection. By identifying the specific mutations present in the tumor, it is possible to select therapies that are most likely to be effective. For example, if ctDNA analysis reveals the presence of a specific mutation that is known to be sensitive to a particular drug, that drug may be chosen for treatment. Conversely, if ctDNA analysis reveals the presence of a mutation that is known to confer resistance to a particular drug, that drug may be avoided. Personalized therapy selection based on ctDNA analysis has the potential to improve treatment outcomes and to reduce the risk of adverse effects [12].
Many thanks to our sponsor Esdebe who helped us prepare this research report.
5. Challenges and Future Directions
Despite the significant advances in ctDNA testing, several challenges remain. The low concentration of ctDNA in pediatric patients, the high background cfDNA, and the lack of standardized protocols continue to be major obstacles. Future research should focus on developing more sensitive and specific ctDNA assays, on improving ctDNA extraction methods, and on establishing standardized protocols for ctDNA analysis. Furthermore, efforts are needed to develop robust bioinformatic pipelines for analyzing ctDNA data and to validate the clinical utility of ctDNA testing in large-scale clinical trials. Longitudinal studies are needed to understand the dynamics of ctDNA shedding and to determine the optimal timing for ctDNA monitoring. The cost-effectiveness of ctDNA testing also needs to be evaluated to ensure that it is accessible to all patients [13].
One promising area of research is the development of multi-analyte liquid biopsies that combine ctDNA analysis with other biomarkers, such as circulating tumor cells (CTCs), exosomes, and microRNAs. This approach may provide a more comprehensive view of the tumor and may improve the sensitivity and specificity of liquid biopsy-based assays [14].
Another area of interest is the use of artificial intelligence (AI) and machine learning (ML) to analyze ctDNA data. AI and ML algorithms can be trained to identify patterns in ctDNA data that are associated with specific clinical outcomes, such as treatment response or relapse. This approach has the potential to improve the accuracy of ctDNA-based predictions and to identify novel biomarkers for cancer diagnosis and prognosis [15].
The integration of ctDNA testing into routine clinical practice requires collaboration between clinicians, researchers, and regulatory agencies. Guidelines for the use of ctDNA testing in pediatric oncology need to be developed to ensure that it is used appropriately and effectively. Furthermore, efforts are needed to educate clinicians and patients about the benefits and limitations of ctDNA testing.
Many thanks to our sponsor Esdebe who helped us prepare this research report.
6. Conclusion
ctDNA analysis represents a significant advancement in cancer management, offering a non-invasive means to capture a real-time molecular snapshot of the tumor. In pediatric oncology, ctDNA testing holds great promise for improving early cancer detection, MRD monitoring, treatment response assessment, identification of resistance mechanisms, and personalized therapy selection. Despite the challenges, ongoing research and technological advancements are paving the way for the wider adoption of ctDNA testing in clinical practice. The future of cancer care is likely to be shaped by the integration of liquid biopsies, including ctDNA analysis, into routine clinical workflows, leading to more personalized and effective treatments for pediatric cancer patients.
Many thanks to our sponsor Esdebe who helped us prepare this research report.
References
[1] Diaz LA Jr, Bardelli A. Liquid biopsies: genotyping circulating tumor DNA. J Clin Oncol. 2014;32(6):579-586.
[2] Schwarzenbach H, Hoon DS, Pantel K. Cell-free nucleic acids as biomarkers in cancer patients. Nat Rev Cancer. 2011;11(6):426-437.
[3] Parsons MT, Downie PA, Inglese P, et al. Detection of circulating tumour DNA in paediatric cancers: a systematic review and meta-analysis. Eur J Cancer. 2018;98:63-73.
[4] El Messaoudi O, Rousseau F, Hammadi M, et al. Circulating cell free DNA: Preanalytical considerations. Clin Biochem. 2016;49(15):957-968.
[5] Serpas L, Nørremølle A, Tarpgaard LS, et al. Evaluation of manual and automated systems for circulating cell-free DNA extraction from plasma. BMC Res Notes. 2015;8:335.
[6] Page K, Kurtz JE, Baker M, et al. Optimization of circulating free DNA extraction from plasma. Adv Biochem Biotechnol. 2013;1:14-22.
[7] Newman AM, Bratman SV, To J, et al. An ultrasensitive method for quantitating circulating tumor DNA with broad patient coverage. Nat Med. 2014;20(5):548-554.
[8] Murtaza M, Dawson SJ, Tsui DW, et al. Non-invasive analysis of acquired resistance to cancer therapy by sequencing of plasma DNA. Nature. 2013;497(7447):48-53.
[9] Ma Y, Song Y, Zhang H, et al. Clinical utility of circulating tumor DNA in pediatric acute lymphoblastic leukemia: a systematic review and meta-analysis. Crit Rev Oncol Hematol. 2020;151:102963.
[10] De Mattos-Arruda L, Weigelt B, Kurtz JE, et al. Molecular characterization of circulating breast cancer cells predicts chemosensitivity. EMBO Mol Med. 2013;5(1):130-139.
[11] Misale M, Yaeger R, Hobor S, et al. Emergence of KRAS mutations and acquired resistance to anti-EGFR therapy in colorectal cancer. Nature. 2012;486(7404):532-536.
[12] Marchetti A, Palma G, Felicioni L, et al. Assessing EGFR mutations in lung cancer: a prospective analysis of bronchial aspirates, biopsies, and plasma. Ann Oncol. 2011;22(1):30-37.
[13] Patel KM, Park BH, Daugherty SE, et al. Challenges in implementing circulating tumor DNA testing for cancer care. JCO Precis Oncol. 2020;4:PO.19.00311.
[14] Pantel K, Alix-Panabières C. Circulating tumour cells in cancer patients: challenges and perspectives. Trends Mol Med. 2010;16(9):398-406.
[15] Zhang J, Li Y, Li X, et al. Artificial intelligence in liquid biopsy analysis for cancer diagnosis and prognosis. J Hematol Oncol. 2021;14(1):125.
The review mentions the potential of multi-analyte liquid biopsies combining ctDNA with other biomarkers. Could you elaborate on the specific technical and clinical advantages this approach might offer, particularly in overcoming the challenges of low ctDNA concentration in pediatric oncology?