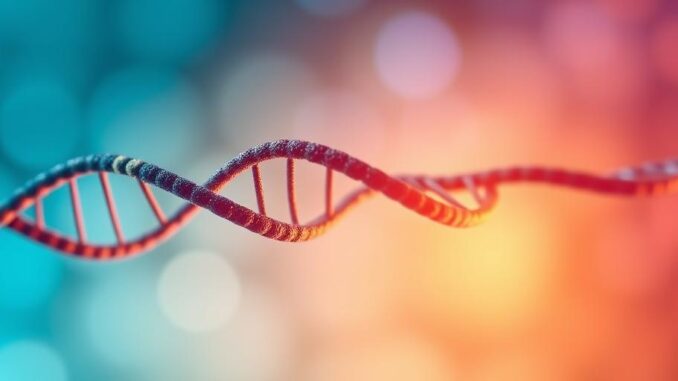
Abstract
CRISPR-Cas systems, originating as adaptive immune mechanisms in bacteria and archaea, have rapidly evolved into a powerful and versatile platform for genome engineering. While the initial impact of CRISPR was most profoundly felt in precise and efficient gene editing, the ongoing revolution extends far beyond simple gene knockouts or insertions. This research report delves into the current state of CRISPR technology, examining not only the established CRISPR-Cas9 and Cas12a systems but also exploring the burgeoning landscape of diverse Cas variants, base editors, prime editors, and CRISPR-based diagnostics. We analyze the advancements in delivery strategies, including both viral and non-viral methods, and address the critical challenges of specificity and off-target effects. Furthermore, this report moves beyond therapeutic applications to investigate the broader implications of CRISPR in agriculture, synthetic biology, and fundamental research. The ethical and regulatory considerations surrounding CRISPR technology, particularly in the context of human germline editing and the potential for unintended consequences, are critically examined. Finally, we provide a perspective on the future trajectory of CRISPR, highlighting emerging trends and potential breakthroughs that will shape the next generation of genome engineering.
Many thanks to our sponsor Esdebe who helped us prepare this research report.
1. Introduction
The discovery and subsequent engineering of CRISPR-Cas systems represent a watershed moment in the history of biotechnology. Initially recognized as repetitive DNA sequences in bacterial genomes, CRISPR (Clustered Regularly Interspaced Short Palindromic Repeats) and their associated Cas (CRISPR-associated) proteins were soon identified as a sophisticated adaptive immune system protecting prokaryotes from invading viruses and plasmids [1, 2]. This system, evolved over billions of years, allows bacteria to memorize past infections and subsequently target and destroy matching genetic material. The inherent programmability of CRISPR-Cas, achieved by simply changing the guide RNA sequence to target a specific DNA locus, has democratized genome editing, making it accessible to researchers worldwide.
The first-generation CRISPR-Cas9 system, derived from Streptococcus pyogenes, quickly became the workhorse of genome editing due to its simplicity and efficiency [3]. However, its limitations, including a requirement for a specific Protospacer Adjacent Motif (PAM) sequence and potential off-target activity, spurred the development of alternative CRISPR systems, each possessing unique characteristics and advantages. This report will expand beyond the well-documented CRISPR-Cas9 system to provide a holistic overview of the CRISPR landscape, encompassing a variety of Cas proteins, delivery methods, and applications.
Many thanks to our sponsor Esdebe who helped us prepare this research report.
2. CRISPR-Cas Systems: A Diverse Toolkit
While CRISPR-Cas9 remains widely utilized, the CRISPR toolbox has expanded dramatically. Understanding the distinct characteristics of different Cas proteins is crucial for selecting the optimal tool for a specific application.
2.1. CRISPR-Cas9: The Pioneer
The CRISPR-Cas9 system, as originally described, utilizes a single Cas9 protein guided by a single guide RNA (sgRNA) composed of a CRISPR RNA (crRNA) and a trans-activating crRNA (tracrRNA). The sgRNA directs Cas9 to a specific DNA sequence complementary to the crRNA, provided that the target sequence is adjacent to a PAM sequence (NGG for S. pyogenes Cas9) [3]. Cas9 then induces a double-strand break (DSB) in the DNA. These DSBs are subsequently repaired by one of two primary cellular mechanisms: non-homologous end joining (NHEJ), which is error-prone and often results in insertions or deletions (indels), or homology-directed repair (HDR), which utilizes a provided DNA template to repair the break with a desired sequence [4]. The efficiency and simplicity of CRISPR-Cas9 made it rapidly adopted for gene knockouts, gene editing, and gene tagging.
2.2. CRISPR-Cas12a (Cpf1): Expanding the Targeting Range
CRISPR-Cas12a (formerly Cpf1) offers several advantages over Cas9. First, it requires a shorter PAM sequence (typically 5′-TTTN-3′), expanding the targeting range [5]. Second, Cas12a processes its own crRNA from a precursor RNA, simplifying the guide RNA design. Third, Cas12a generates staggered DNA breaks, which can enhance the efficiency of HDR and reduce off-target effects compared to Cas9 [6]. The staggered cuts promote more efficient HDR and alter the kinetics of the repair machinery reducing opportunities for NHEJ-induced errors. The Cas12a-based RGN (RNA-guided endonuclease) is also smaller than Cas9, facilitating delivery in vivo, especially when using adeno-associated virus (AAV) vectors with limited cargo capacity.
2.3. Other CRISPR-Cas Systems: A Growing Family
The discovery of numerous other CRISPR-Cas systems, including Cas13, Cas14, and CasX, has further expanded the capabilities of genome engineering. Cas13 targets RNA instead of DNA, making it valuable for RNA knockdown, RNA editing, and RNA-based diagnostics [7]. Cas14 is a smaller Cas protein that can target single-stranded DNA, expanding targeting scope for diagnostic and therapeutic applications [8]. CasX is a structurally distinct CRISPR effector with a compact size and novel mechanism of action, which may further reduce off-target effects and improve delivery [9]. Ongoing research continues to uncover novel CRISPR-Cas systems with unique functionalities, solidifying CRISPR as a rapidly evolving and highly adaptable platform.
2.4. Deactivated Cas (dCas) and Base Editors: Expanding Functionality beyond Cutting
Catalytically inactive or ‘dead’ Cas9 (dCas9) and dCas12a retain their ability to bind to specific DNA sequences but lack the ability to cleave the DNA. These dCas proteins can be fused to other functional domains, such as transcriptional activators or repressors, to modulate gene expression [10]. This approach allows for targeted regulation of gene expression without permanently altering the DNA sequence, offering a reversible and precise method for controlling cellular processes.
Base editors represent a further advancement. These innovative tools fuse a catalytically inactive Cas protein with a deaminase enzyme, which can directly convert one DNA base pair into another (e.g., C•G to T•A or A•T to G•C) without creating a DSB [11]. Base editors offer high precision and reduced off-target effects compared to traditional CRISPR-Cas9, making them attractive for therapeutic applications where precise sequence correction is paramount.
2.5. Prime Editing: A Versatile and Precise Editing Tool
Prime editing, a more recent development, utilizes a Cas9 nickase (H840A mutant) fused to a reverse transcriptase enzyme and a prime editing guide RNA (pegRNA) [12]. The pegRNA contains both a guide sequence for targeting and a reverse transcriptase template for encoding the desired edit. Prime editing allows for precise insertion, deletion, and base substitution without requiring a DSB or a donor DNA template, offering unparalleled versatility and precision. While prime editing is generally less efficient than CRISPR-Cas9 for gene knockouts, its ability to perform complex edits with minimal off-target effects makes it a powerful tool for therapeutic applications.
Many thanks to our sponsor Esdebe who helped us prepare this research report.
3. Delivery Strategies for CRISPR-Cas Systems
Efficient and safe delivery of CRISPR-Cas components into target cells or tissues is crucial for both in vitro and in vivo applications. Delivery methods can be broadly classified as viral and non-viral.
3.1. Viral Vectors: High Transduction Efficiency
Viral vectors, particularly adeno-associated viruses (AAVs) and lentiviruses, are widely used for CRISPR-Cas delivery due to their high transduction efficiency. AAVs are small, non-integrating viruses with low immunogenicity, making them suitable for in vivo gene therapy [13]. However, AAVs have a limited packaging capacity, which can be a constraint for delivering larger Cas proteins like Cas9. Lentiviruses, on the other hand, can accommodate larger payloads and can integrate into the host cell genome, providing long-term expression of the CRISPR-Cas components [14]. However, the risk of insertional mutagenesis associated with lentiviruses must be carefully considered. AAV serotype selection is a critical consideration when choosing a delivery strategy because different AAV serotypes exhibit tropism for distinct tissues. Selecting the most appropriate serotype increases the likelihood of successful targeted delivery.
3.2. Non-Viral Delivery: Lower Immunogenicity and Flexibility
Non-viral delivery methods, such as electroporation, lipofection, and nanoparticle-mediated delivery, offer several advantages over viral vectors, including lower immunogenicity and greater flexibility in payload design. Electroporation involves the use of brief electrical pulses to create transient pores in the cell membrane, allowing the entry of CRISPR-Cas components [15]. Lipofection utilizes lipid-based carriers to encapsulate and deliver CRISPR-Cas components into cells [16]. Nanoparticle-mediated delivery, including lipid nanoparticles (LNPs) and polymer-based nanoparticles, offers targeted delivery and controlled release of CRISPR-Cas components [17]. LNPs are particularly attractive for in vivo delivery due to their biocompatibility and ability to efficiently deliver mRNA encoding Cas proteins.
Each delivery method has its own advantages and disadvantages in terms of efficiency, specificity, and immunogenicity. The choice of delivery method depends on the specific application, the target cell type, and the desired duration of CRISPR-Cas activity. Optimization of delivery parameters is often required to achieve optimal editing efficiency and minimize off-target effects.
Many thanks to our sponsor Esdebe who helped us prepare this research report.
4. Specificity and Off-Target Effects
One of the major challenges associated with CRISPR-Cas technology is the potential for off-target effects, where the Cas protein cleaves DNA at unintended sites in the genome. Off-target effects can lead to unintended mutations and potentially harmful consequences, such as cancer development. Understanding and mitigating off-target effects is crucial for the safe and effective application of CRISPR-Cas technology.
4.1. Factors Influencing Off-Target Activity
Several factors can influence the specificity of CRISPR-Cas systems, including the guide RNA sequence, the Cas protein variant, the concentration of CRISPR-Cas components, and the chromatin accessibility of the target site. Guide RNAs with high homology to off-target sites are more likely to induce off-target cleavage [18]. Some Cas protein variants, such as SpCas9-HF1 and eSpCas9, have been engineered to reduce off-target activity by increasing their stringency for target binding [19, 20]. Lowering the concentration of CRISPR-Cas components can also reduce off-target effects, although this may also decrease on-target efficiency. Areas of open chromatin are generally more susceptible to CRISPR-Cas cleavage than regions of condensed chromatin.
4.2. Strategies for Minimizing Off-Target Effects
A variety of strategies have been developed to minimize off-target effects, including:
- Rational guide RNA design: Using bioinformatics tools to identify and avoid guide RNAs with high homology to potential off-target sites.
- Truncated guide RNAs: Shortening the guide RNA sequence to increase specificity.
- Paired nickases: Using two Cas9 nickases (H840A mutant) to create a DSB only when both nickases bind near each other, significantly reducing off-target cleavage.
- High-fidelity Cas variants: Using engineered Cas proteins with increased specificity, such as SpCas9-HF1, eSpCas9, and evoCas9.
- Delivery optimization: Optimizing delivery parameters to minimize the concentration of CRISPR-Cas components.
- Off-target detection methods: Utilizing unbiased genome-wide off-target detection methods, such as GUIDE-seq, Digenome-seq, and CIRCLE-seq, to identify and characterize off-target cleavage sites [21, 22, 23].
4.3. The Role of Genome-Wide Off-Target Detection
Unbiased genome-wide off-target detection methods are crucial for comprehensively assessing the specificity of CRISPR-Cas systems and identifying potential off-target cleavage sites. These methods can help to guide guide RNA design, optimize delivery parameters, and select appropriate Cas protein variants. The ideal strategy will vary based on the model used, but the general consensus is to use at least two independent methods of off-target detection to confirm that on-target effects outweigh potential off-target activities.
Many thanks to our sponsor Esdebe who helped us prepare this research report.
5. Applications of CRISPR Technology
The versatility of CRISPR technology has led to its widespread adoption in a wide range of applications, including gene therapy, agriculture, synthetic biology, and fundamental research.
5.1. Gene Therapy: Correcting Genetic Defects
CRISPR-Cas systems hold tremendous promise for treating genetic diseases by correcting or disrupting disease-causing genes. Several CRISPR-based gene therapies are currently in clinical trials for diseases such as sickle cell disease, beta-thalassemia, Duchenne muscular dystrophy, and inherited blindness [24]. These therapies typically involve either ex vivo editing of patient-derived cells followed by transplantation or in vivo delivery of CRISPR-Cas components to target tissues. While early clinical trial results are promising, long-term safety and efficacy data are still needed. Gene therapy remains one of the most promising therapeutic areas that will be revolutionized by advances in CRISPR technology.
5.2. Agriculture: Improving Crop Yield and Resistance
CRISPR technology is being used to improve crop yield, enhance nutritional content, and increase resistance to pests and diseases. CRISPR-edited crops are being developed with traits such as increased grain size, improved drought tolerance, and resistance to fungal pathogens [25]. CRISPR-based genome editing offers a precise and efficient alternative to traditional plant breeding methods, accelerating the development of improved crop varieties. The application of CRISPR technology in agriculture has the potential to address global food security challenges and reduce the environmental impact of agriculture. The relative ease and speed of making changes compared to conventional breeding strategies allows for the use of less land, less water, and fewer resources to feed the world.
5.3. Synthetic Biology: Engineering Biological Systems
CRISPR technology is a powerful tool for synthetic biology, enabling the precise engineering of biological systems for a variety of applications, including the production of biofuels, pharmaceuticals, and biomaterials. CRISPR-Cas systems can be used to create synthetic gene circuits, modify metabolic pathways, and design novel biological functions [26]. The ability to precisely manipulate the genome allows for the creation of complex and sophisticated biological systems with tailored functionalities. The programmable nature of CRISPR promotes the creation of designer circuits for use as biosensors to monitor environmental variables or for use as biological factories to produce valuable biochemicals such as fuels, flavors, and materials.
5.4. Fundamental Research: Unraveling Biological Mechanisms
CRISPR technology is a valuable tool for fundamental research, allowing scientists to study gene function, regulatory networks, and disease mechanisms. CRISPR-Cas systems can be used to create gene knockouts, gene knock-ins, and gene editing models to investigate the role of specific genes in cellular processes [27]. CRISPR-based screening approaches allow for the systematic identification of genes involved in various biological pathways and disease processes. This acceleration of discovery allows researchers to more rapidly identify drug targets and begin to understand the complexities of human biology.
Many thanks to our sponsor Esdebe who helped us prepare this research report.
6. Ethical and Regulatory Considerations
The immense potential of CRISPR technology also raises significant ethical and regulatory considerations, particularly in the context of human germline editing and the potential for unintended consequences.
6.1. Human Germline Editing: A Contentious Issue
Human germline editing, which involves making changes to the DNA of sperm, eggs, or early embryos, is a highly contentious issue due to the potential for these changes to be inherited by future generations. While germline editing could potentially eradicate inherited genetic diseases, it also raises concerns about unintended consequences, the potential for misuse, and the long-term impact on the human gene pool [28]. Many scientists and ethicists argue that germline editing should be prohibited or strictly regulated until the safety and ethical implications are thoroughly understood.
6.2. Regulatory Landscape of CRISPR-Based Therapies
The regulatory landscape of CRISPR-based therapies is still evolving. In many countries, CRISPR-based therapies are regulated as gene therapies, requiring rigorous preclinical and clinical testing to ensure safety and efficacy. The regulatory pathways for CRISPR-edited crops and livestock are also under development. The development of clear and consistent regulatory frameworks is essential for fostering innovation while ensuring responsible development and use of CRISPR technology. A lack of global consistency in regulations can create challenges for companies and researchers, potentially slowing down the development and accessibility of these therapies.
6.3. Informed Consent and Public Engagement
Informed consent is a critical ethical consideration in the clinical application of CRISPR technology. Patients participating in CRISPR-based clinical trials must fully understand the potential risks and benefits of the treatment, as well as the limitations of the technology. Public engagement is also essential for fostering informed discussions about the ethical and societal implications of CRISPR technology and for shaping responsible policies and regulations. The public needs to be well-informed to avoid misconceptions about the technology’s capabilities and to participate in meaningful discussions about its future.
Many thanks to our sponsor Esdebe who helped us prepare this research report.
7. Future Directions and Concluding Remarks
CRISPR technology has revolutionized genome engineering and continues to evolve at a rapid pace. Future directions for CRISPR research include:
- Developing more precise and efficient CRISPR-Cas systems: Engineering Cas proteins with improved specificity and reduced off-target effects.
- Improving delivery methods: Developing more efficient and targeted delivery methods for CRISPR-Cas components.
- Expanding the CRISPR toolbox: Discovering and characterizing novel CRISPR-Cas systems with unique functionalities.
- Exploring new applications of CRISPR technology: Applying CRISPR technology to address pressing challenges in medicine, agriculture, and environmental sustainability.
- Addressing ethical and regulatory challenges: Developing ethical guidelines and regulatory frameworks that promote responsible innovation and ensure the safe and equitable use of CRISPR technology.
The future of CRISPR technology is bright. While challenges remain, the potential benefits of CRISPR for treating diseases, improving crop production, and advancing our understanding of biology are immense. Continued research and responsible development of CRISPR technology will pave the way for a future where genome engineering can be used to improve human health and well-being.
Concluding Remarks
From its origins as a bacterial defense mechanism, CRISPR has rapidly transformed into an indispensable tool for genome engineering. The journey has seen an explosion of innovation, extending far beyond the initial CRISPR-Cas9 system to encompass diverse Cas variants, sophisticated editing tools like base and prime editors, and a growing understanding of delivery mechanisms and off-target effects. While therapeutic applications hold enormous promise, the broader impact of CRISPR is already being felt in agriculture, synthetic biology, and fundamental research. As the technology continues to mature, the ethical and regulatory considerations surrounding germline editing and responsible innovation demand careful attention and open dialogue. The future of CRISPR hinges on a commitment to rigorous research, responsible development, and proactive engagement with the ethical and societal implications of this powerful technology. Only through this collaborative approach can we unlock the full potential of CRISPR to improve human health and well-being while safeguarding against potential risks.
Many thanks to our sponsor Esdebe who helped us prepare this research report.
References
[1] Ishino, Y., et al. (1987). Nucleotide sequence of the iap gene, responsible for alkaline phosphatase isoenzyme conversion in Escherichia coli, and identification of the gene product. Journal of Bacteriology, 169(12), 5429-5433.
[2] Jansen, R., et al. (2002). Identification of genes that are associated with DNA repeats in prokaryotes. Molecular Microbiology, 43(6), 1565-1575.
[3] Jinek, M., et al. (2012). A programmable dual-RNA-guided DNA endonuclease in adaptive bacterial immunity. Science, 337(6096), 816-821.
[4] Heyer, W. D., Ehmsen, K. T., & Liu, J. (2010). Regulation of homologous recombination in eukaryotes. Annual Review of Genetics, 44, 39-68.
[5] Zetsche, B., et al. (2015). Cpf1 is a single RNA-guided endonuclease of a class 2 CRISPR-Cas system. Cell, 163(3), 759-771.
[6] Kim, D., et al. (2016). Genome-wide analysis reveals specificities of CRISPR-Cas9 endonucleases. Nature Biotechnology, 34(8), 863-869.
[7] East-Seletsky, A., et al. (2016). Two distinct classes of prokaryotic Argonaute proteins with guide-dependent nuclease activity. Nature, 538(7624), 257-261.
[8] Harrington, L. B., et al. (2018). Programmed DNA destruction by miniature CRISPR-Cas14 enzymes. Science, 362(6416), 839-842.
[9] Liu, J. J., et al. (2019). CasX enzymes comprise a distinct family of CRISPR-Cas systems. Nature, 566(7743), 218-223.
[10] Gilbert, L. A., et al. (2013). CRISPR-mediated modular RNA-guided regulation of transcription in eukaryotes. Cell, 154(2), 442-451.
[11] Komor, A. C., Kim, Y. B., Packer, M. S., Zuris, J. A., & Liu, D. R. (2016). Programmable editing of a target base in genomic DNA without double-stranded DNA cleavage. Nature, 533(7603), 420-424.
[12] Anzalone, A. V., et al. (2019). Search-and-replace genome editing without double-strand breaks or donor DNA. Nature, 576(7785), 149-157.
[13] Naso, M. F., Tomkowicz, B., Perry, W. L. III, & Strohl, W. R. (2017). Adeno-associated virus (AAV) as a vector for gene therapy. BioDrugs, 31(4), 317-334.
[14] Cavazzana-Calvo, M., et al. (2000). Gene therapy of human severe combined immunodeficiency (SCID)-X1 disease. Science, 288(5466), 669-672.
[15] Neumann, E., Schaefer-Ridder, M., Wang, Y., & Hofschneider, P. H. (1982). Gene transfer into mouse lyoma cells by electroporation in high electric fields. The EMBO Journal, 1(7), 841-845.
[16] Felgner, P. L., Gadek, T. R., Holm, M., Roman, R., Chan, H. W., Wenz, M., Northrop, J. P., Ringold, G. M., & Danielsen, M. (1987). Lipofection: a highly efficient, lipid-mediated DNA-transfection procedure. Proceedings of the National Academy of Sciences, 84(21), 7413-7417.
[17] Anselmo, A. C., & Mitragotri, S. (2016). Nanoparticles in drug delivery. Bioorganic & Medicinal Chemistry, 24(10), 1063-1070.
[18] Hsu, P. D., et al. (2013). DNA targeting specificity of RNA-guided Cas9 nucleases. Nature Biotechnology, 31(9), 827-832.
[19] Slaymaker, I. M., et al. (2016). Rationally engineered Cas9 nucleases with improved specificity. Science, 351(6268), 84-88.
[20] Kleinstiver, B. P., et al. (2016). High-fidelity CRISPR-Cas9 nucleases with no detectable off-target activity. Nature, 529(7587), 490-495.
[21] Tsai, S. Q., et al. (2015). GUIDE-seq: a genome-wide unbiased identification of DNA off-target effects mediated by CRISPR-Cas systems. Nature Biotechnology, 33(2), 187-197.
[22] Kim, D., Bae, S., Park, J., Kim, J., & Kim, J. S. (2015). Digenome-seq: genome-wide profiling of CRISPR-on-target and off-target effects using sequencing depth. Nature Methods, 12(3), 237-243.
[23] Tsai, S. Q., et al. (2017). CIRCLE-seq: a highly sensitive and unbiased method for genome-wide off-target detection. Nature Methods, 14(6), 607-614.
[24] Ledford, H. (2020). CRISPR gene-editing tested in human patients for the first time. Nature, 578(7794), 197-198.
[25] Zhang, H., & Gao, C. (2016). CRISPR-Cas9 technology is revolutionizing crop breeding. Nature Plants, 2(11), 1-6.
[26] Khalil, A. S., & Collins, J. J. (2010). Synthetic biology: applications come of age. Nature Reviews Genetics, 11(5), 367-379.
[27] Doudna, J. A., & Charpentier, E. (2014). Genome editing. The new frontier of genome engineering with CRISPR-Cas9. Science, 346(6213), 1258096.
[28] Lander, E. S. (2016). The heroes of CRISPR. Cell, 164(1-2), 1-12.
The discussion on ethical and regulatory considerations is critical. What specific challenges do you foresee in achieving international consensus on guidelines for human germline editing, given the varying cultural and legal perspectives?
Great point! Achieving international consensus will be tough. Varying cultural and legal perspectives on autonomy, disability, and the role of technology in shaping future generations will present substantial obstacles. Open dialogue and education are crucial to bridging these divides. Thanks for raising this important issue!
Editor: MedTechNews.Uk
Thank you to our Sponsor Esdebe
The report highlights the expanding CRISPR toolbox. How do factors like cost, ease of use, and intellectual property rights influence the adoption of newer CRISPR systems (e.g., Cas12a, Cas13, prime editing) compared to the more established CRISPR-Cas9, particularly in smaller research labs or developing countries?
That’s a fantastic question! The interplay of cost, ease of use, and IP is definitely a key factor. We’re seeing innovative licensing models emerge that could help make these newer CRISPR systems more accessible to smaller labs. Perhaps this may democratise the accessibility of CRISPR tools. What are your thoughts on this point?
Editor: MedTechNews.Uk
Thank you to our Sponsor Esdebe
This report comprehensively highlights the advancements in CRISPR technology. The discussion of delivery strategies, particularly non-viral methods like LNPs for mRNA delivery of Cas proteins, is interesting. Further research into optimizing these methods could significantly improve the safety profile of in vivo CRISPR applications.
Thank you for your insightful comment. I agree that optimizing non-viral delivery methods, especially LNPs, is crucial. The improved safety profile could open doors for more widespread in vivo CRISPR applications and ultimately benefit patients. Exploring targeted LNPs could be a game changer, what are your thoughts on targeting methods?
Editor: MedTechNews.Uk
Thank you to our Sponsor Esdebe
The ethical considerations are indeed critical! I wonder, if CRISPR is used to enhance traits (think super eyesight), how do we ensure equitable access and prevent a genetic divide, creating a literal “haves” and “have-nots”?
That’s a really important point. The potential for trait enhancement raises serious questions about fairness and access. Thinking ahead, perhaps a framework for tiered access, prioritizing treatments for genetic diseases while carefully evaluating enhancement requests, could be part of the solution? It’s a complex issue that demands open discussion. Thanks for bringing this up!
Editor: MedTechNews.Uk
Thank you to our Sponsor Esdebe