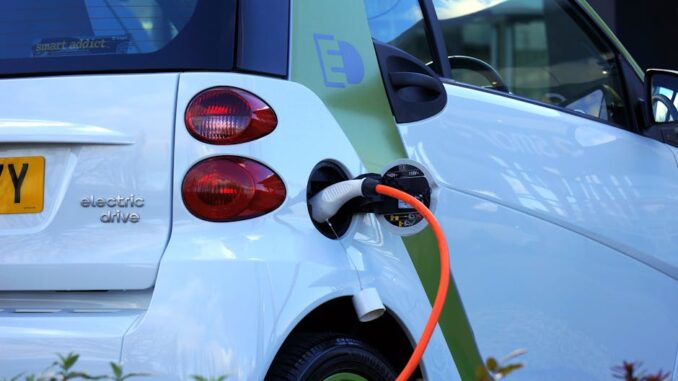
Abstract
Lithium-ion batteries (LIBs) have revolutionized portable electronics and are increasingly vital for electric vehicles and grid-scale energy storage. The electrolyte, acting as the ionically conductive medium between the electrodes, critically governs LIB performance, safety, and lifespan. This comprehensive review explores the multifaceted role of the electrolyte, encompassing its fundamental properties (ionic conductivity, viscosity, transference number, electrochemical stability window), composition (liquid, solid-state, redox-active, localized high concentration), and interactions with electrode materials. We delve into the influence of electrolyte properties on key performance metrics, including rate capability, coulombic efficiency, and cycling stability. Furthermore, we examine the degradation mechanisms mediated by electrolyte decomposition, metal ion dissolution, and dendrite formation, proposing mitigation strategies based on advanced electrolyte design. Finally, we discuss emerging electrolyte chemistries and architectures, highlighting their potential to address the limitations of conventional LIBs and enable next-generation energy storage technologies. The review also offers a critical perspective on the challenges and opportunities in electrolyte engineering for achieving high-performance, safe, and durable LIBs.
Many thanks to our sponsor Esdebe who helped us prepare this research report.
1. Introduction
The relentless demand for high-performance energy storage systems has propelled the development of lithium-ion batteries (LIBs) to the forefront of scientific and technological innovation. From powering portable electronics to enabling electric vehicles and grid-scale energy storage, LIBs have become indispensable components of modern society [1]. A pivotal element within the LIB architecture is the electrolyte, which serves as the ionic pathway facilitating the transport of lithium ions between the cathode and anode during charge and discharge cycles. The electrolyte’s properties, composition, and interactions with the electrode materials profoundly influence the overall performance, safety, and longevity of the battery [2].
Conventional LIBs predominantly employ liquid electrolytes, typically comprising lithium salts dissolved in organic solvents. While offering high ionic conductivity, these electrolytes suffer from inherent limitations, including flammability, narrow electrochemical stability windows, and susceptibility to decomposition [3]. These shortcomings contribute to capacity fade, dendrite formation, and thermal runaway, posing significant challenges to the widespread adoption of LIBs, particularly in safety-critical applications such as electric vehicles. Consequently, extensive research efforts are directed towards developing advanced electrolyte chemistries and architectures that can overcome these limitations and enhance the performance and safety of LIBs [4].
This review aims to provide a comprehensive overview of the electrolyte landscape in LIBs, encompassing its fundamental properties, composition, and interactions with electrode materials. We will explore the influence of electrolyte properties on key performance metrics, including rate capability, coulombic efficiency, and cycling stability. Furthermore, we will delve into the degradation mechanisms mediated by electrolyte decomposition, metal ion dissolution, and dendrite formation, proposing mitigation strategies based on advanced electrolyte design. Finally, we will discuss emerging electrolyte chemistries and architectures, highlighting their potential to address the limitations of conventional LIBs and enable next-generation energy storage technologies.
Many thanks to our sponsor Esdebe who helped us prepare this research report.
2. Fundamental Electrolyte Properties
The performance of an electrolyte within a LIB is fundamentally determined by its key properties. These properties, including ionic conductivity, viscosity, transference number, electrochemical stability window (ESW), and thermal stability, dictate the electrolyte’s ability to efficiently transport lithium ions, resist degradation, and maintain compatibility with the electrode materials [5].
2.1 Ionic Conductivity
Ionic conductivity (σ) is arguably the most critical electrolyte property, representing the ease with which lithium ions can migrate through the electrolyte. A high ionic conductivity minimizes the internal resistance of the battery, enabling high rate capability and efficient energy delivery [6]. In liquid electrolytes, ionic conductivity is influenced by factors such as the concentration and mobility of lithium ions, the dielectric constant and viscosity of the solvent, and the dissociation constant of the lithium salt. The relationship between ionic conductivity and these factors can be described by the Nernst-Einstein equation [7]. Typical liquid electrolytes exhibit ionic conductivities in the range of 1-10 mS/cm at room temperature. However, solid-state electrolytes generally have lower ionic conductivities, often in the range of 10-6 to 10-3 S/cm, although recent advancements are closing this gap [8].
2.2 Viscosity
Viscosity (η) is a measure of a fluid’s resistance to flow. In LIB electrolytes, viscosity affects the ion mobility and diffusion rate. High viscosity can hinder ion transport, reducing ionic conductivity and limiting rate performance [9]. Conversely, excessively low viscosity can lead to electrolyte leakage and increased flammability. Balancing viscosity is crucial for optimal electrolyte performance. For instance, the use of co-solvents or additives can modulate the viscosity of liquid electrolytes to achieve a desired balance between ionic conductivity and safety [10].
2.3 Lithium-Ion Transference Number
The lithium-ion transference number (tLi+) represents the fraction of the total ionic current carried by lithium ions. Ideally, tLi+ should be close to unity, indicating that almost all the current is carried by lithium ions, minimizing concentration polarization and maximizing battery performance [11]. In reality, however, most liquid electrolytes have tLi+ values significantly less than unity, due to the presence of anionic species that also contribute to the ionic current. Strategies to enhance tLi+ include the use of single-ion conducting electrolytes or the incorporation of additives that selectively immobilize anions [12].
2.4 Electrochemical Stability Window
The electrochemical stability window (ESW) defines the voltage range within which the electrolyte is electrochemically stable and does not undergo oxidation or reduction reactions. A wide ESW is essential for compatibility with high-voltage cathode materials and prevents electrolyte decomposition at high potentials, which can lead to capacity fade and gas generation [13]. Liquid electrolytes based on organic solvents often have limited ESWs, typically below 4.5 V vs. Li/Li+. The addition of film-forming additives, such as vinylene carbonate (VC) and fluoroethylene carbonate (FEC), can extend the ESW by forming a protective solid electrolyte interphase (SEI) layer on the anode surface [14].
2.5 Thermal Stability
Thermal stability is a crucial safety consideration for LIB electrolytes. Electrolytes with poor thermal stability can decompose at elevated temperatures, generating flammable gases and potentially leading to thermal runaway [15]. The thermal stability of an electrolyte is influenced by its chemical composition and the presence of impurities. Additives such as flame retardants can improve the thermal stability of liquid electrolytes. Solid-state electrolytes generally exhibit superior thermal stability compared to liquid electrolytes, enhancing the safety of LIBs [16].
Many thanks to our sponsor Esdebe who helped us prepare this research report.
3. Electrolyte Composition and Structure
The composition and structure of the electrolyte play a significant role in determining its properties and interactions with electrode materials. Electrolytes can be broadly classified into liquid, solid-state, redox-active, and localized high concentration electrolytes [17].
3.1 Liquid Electrolytes
Liquid electrolytes are the most commonly used electrolytes in commercial LIBs. They typically consist of a lithium salt dissolved in a mixture of organic solvents. The choice of lithium salt and solvent(s) significantly affects the electrolyte’s ionic conductivity, viscosity, ESW, and thermal stability [18].
3.1.1 Lithium Salts
Commonly used lithium salts include lithium hexafluorophosphate (LiPF6), lithium bis(trifluoromethanesulfonyl)imide (LiTFSI), lithium perchlorate (LiClO4), and lithium tetrafluoroborate (LiBF4). LiPF6 is the most widely used lithium salt due to its high ionic conductivity and relatively low cost [19]. However, LiPF6 is susceptible to hydrolysis, forming hydrofluoric acid (HF), which can corrode the electrode materials and accelerate battery degradation. LiTFSI offers better thermal and electrochemical stability compared to LiPF6, but it can corrode aluminum current collectors at high potentials. LiClO4 exhibits high ionic conductivity but poses a safety hazard due to its explosive nature. LiBF4 is relatively stable and less corrosive than LiPF6 but has lower ionic conductivity [20].
3.1.2 Organic Solvents
Organic solvents used in liquid electrolytes include cyclic carbonates (e.g., ethylene carbonate (EC), propylene carbonate (PC)), linear carbonates (e.g., dimethyl carbonate (DMC), diethyl carbonate (DEC)), and ethers (e.g., dimethoxyethane (DME), tetrahydrofuran (THF)). EC is a commonly used solvent due to its high dielectric constant, which promotes the dissolution of lithium salts [21]. However, EC has a high melting point, which can limit the low-temperature performance of LIBs. DMC and DEC are often used as co-solvents to reduce the viscosity of the electrolyte and improve its low-temperature performance. Ethers offer high ionic conductivity and good compatibility with lithium metal anodes but have low oxidation stability. The choice of solvent mixture is crucial for optimizing the electrolyte’s properties and compatibility with electrode materials [22].
3.2 Solid-State Electrolytes
Solid-state electrolytes (SSEs) have garnered significant attention as promising alternatives to liquid electrolytes due to their non-flammability, wide electrochemical stability windows, and potential to enable the use of lithium metal anodes [23]. SSEs can be classified into inorganic ceramics (e.g., garnet-type oxides, perovskites, NASICON-type materials), polymers (e.g., polyethylene oxide (PEO), polyacrylonitrile (PAN)), and composite electrolytes.
3.2.1 Inorganic Ceramic Electrolytes
Garnet-type oxides, such as Li7La3Zr2O12 (LLZO), exhibit high ionic conductivity and excellent electrochemical stability [24]. However, LLZO is brittle and suffers from poor interfacial contact with the electrodes. Perovskites, such as Li3xLa(2/3)-xTiO3 (LLTO), also show high ionic conductivity but are prone to reduction at low potentials. NASICON-type materials, such as Li1+xAlxTi2-x(PO4)3 (LATP), offer good ionic conductivity and stability but can react with lithium metal anodes [25].
3.2.2 Polymer Electrolytes
Polymer electrolytes, such as PEO, offer flexibility and ease of processing but have low ionic conductivity at room temperature. The ionic conductivity of polymer electrolytes can be improved by adding lithium salts or incorporating plasticizers [26]. However, the mechanical strength and electrochemical stability of polymer electrolytes remain challenges. Composite electrolytes, consisting of a polymer matrix and inorganic fillers, can combine the advantages of both materials, offering improved ionic conductivity and mechanical properties [27].
3.3 Redox-Active Electrolytes
Redox-active electrolytes contain electrochemically active species that can undergo reversible oxidation and reduction reactions, shuttling electrons between the electrodes and compensating for capacity fade [28]. Redox-active electrolytes can also act as overcharge protection devices, preventing the battery from being overcharged. Examples of redox-active species include ferrocene derivatives, quinones, and redox polymers [29].
3.4 Localized High Concentration Electrolytes (LHCEs)
Localized high concentration electrolytes (LHCEs) are a class of electrolytes designed to enhance the lithium-ion transference number and improve the electrochemical stability window. LHCEs achieve this by using a high concentration of lithium salt in a solvent mixture that includes a diluent with weak solvation power. The high salt concentration promotes the formation of lithium-ion aggregates, which reduces anion mobility and increases the lithium-ion transference number [30]. The diluent reduces the overall viscosity of the electrolyte and improves its low-temperature performance. LHCEs have shown promising results in improving the performance and safety of LIBs [31].
Many thanks to our sponsor Esdebe who helped us prepare this research report.
4. Electrolyte Interactions with Electrode Materials
The performance and lifespan of LIBs are significantly influenced by the interactions between the electrolyte and the electrode materials. These interactions can lead to the formation of a solid electrolyte interphase (SEI) layer on the anode surface, metal ion dissolution from the cathode, and dendrite formation on the lithium metal anode [32].
4.1 Solid Electrolyte Interphase (SEI) Formation
The SEI is a passivation layer that forms on the anode surface due to the electrochemical decomposition of the electrolyte. The SEI layer ideally prevents further electrolyte decomposition and stabilizes the anode-electrolyte interface [33]. The composition and properties of the SEI layer significantly affect the performance and lifespan of LIBs. A stable and ionically conductive SEI layer is crucial for maintaining high coulombic efficiency and preventing capacity fade. The formation of the SEI layer is influenced by the electrolyte composition, anode material, and operating conditions [34]. Additives such as VC and FEC are commonly used to promote the formation of a robust and stable SEI layer. Novel SEI formation strategies involve using artificial SEI coatings or pre-lithiation techniques to modify the anode surface [35].
4.2 Metal Ion Dissolution
Metal ion dissolution from the cathode material into the electrolyte can lead to capacity fade and reduced battery performance. Transition metal ions, such as manganese, cobalt, and nickel, can dissolve from the cathode surface due to the presence of HF or other corrosive species in the electrolyte [36]. The dissolved metal ions can migrate to the anode and deposit on the SEI layer, increasing the interfacial resistance and hindering lithium-ion transport. Strategies to mitigate metal ion dissolution include coating the cathode material with a protective layer, using electrolyte additives to scavenge HF, and optimizing the electrolyte composition to minimize the formation of corrosive species [37].
4.3 Dendrite Formation
Dendrite formation on the lithium metal anode is a major safety concern and a significant obstacle to the development of high-energy-density LIBs. During charging, lithium ions can deposit unevenly on the anode surface, leading to the formation of lithium dendrites [38]. These dendrites can grow through the electrolyte and short-circuit the battery, causing thermal runaway and potentially leading to fire or explosion. The formation of lithium dendrites is influenced by the electrolyte composition, surface morphology of the anode, and charging conditions. Strategies to suppress dendrite formation include using solid-state electrolytes, optimizing the electrolyte composition to promote uniform lithium-ion flux, and applying external pressure to the anode [39].
Many thanks to our sponsor Esdebe who helped us prepare this research report.
5. Emerging Electrolyte Chemistries and Architectures
To address the limitations of conventional LIB electrolytes and enable next-generation energy storage technologies, researchers are exploring novel electrolyte chemistries and architectures [40]. Some promising emerging approaches include:
5.1 Ionic Liquids
Ionic liquids (ILs) are salts that are liquid at room temperature. They exhibit high ionic conductivity, non-flammability, and wide electrochemical stability windows, making them attractive candidates for LIB electrolytes [41]. However, ILs typically have high viscosity and poor compatibility with some electrode materials. Researchers are developing modified ILs with improved properties and exploring their use in combination with other electrolyte components [42].
5.2 Deep Eutectic Solvents
Deep eutectic solvents (DESs) are mixtures of two or more compounds that form a eutectic mixture with a melting point significantly lower than that of the individual components. DESs are relatively inexpensive, environmentally friendly, and can be tailored to exhibit specific properties [43]. DESs are being explored as alternative solvents for LIB electrolytes, offering potential advantages in terms of safety and cost [44].
5.3 Single-Ion Conducting Electrolytes
Single-ion conducting electrolytes (SICEs) are designed to transport only lithium ions, eliminating the formation of concentration gradients and maximizing the lithium-ion transference number [45]. SICEs can be based on polymers, inorganic materials, or ionic liquids. The development of SICEs is a promising approach to improving the performance and stability of LIBs [46].
5.4 Electrolyte Additives
Electrolyte additives are small molecules that are added to the electrolyte in small concentrations to improve its properties or modify the electrode-electrolyte interface. Additives can be used to enhance the SEI formation, suppress metal ion dissolution, improve the thermal stability of the electrolyte, or act as redox shuttles [47]. The selection and optimization of electrolyte additives are crucial for achieving high-performance and long-lasting LIBs [48].
Many thanks to our sponsor Esdebe who helped us prepare this research report.
6. Challenges and Opportunities
While significant progress has been made in electrolyte development for LIBs, several challenges remain. Improving the ionic conductivity of solid-state electrolytes, enhancing the lithium-ion transference number of liquid electrolytes, and suppressing dendrite formation on lithium metal anodes are key priorities [49]. Furthermore, developing electrolytes that are compatible with high-voltage cathode materials and operate effectively over a wide temperature range is crucial for expanding the applications of LIBs [50].
Despite these challenges, significant opportunities exist for further advancements in electrolyte technology. The development of novel electrolyte chemistries, such as localized high concentration electrolytes and single-ion conducting electrolytes, holds great promise for improving the performance and safety of LIBs [51]. Furthermore, the use of advanced characterization techniques, such as electrochemical impedance spectroscopy (EIS), nuclear magnetic resonance (NMR), and X-ray photoelectron spectroscopy (XPS), can provide valuable insights into the electrolyte properties and electrode-electrolyte interactions [52]. These insights can guide the design and optimization of next-generation electrolyte materials and architectures.
Many thanks to our sponsor Esdebe who helped us prepare this research report.
7. Conclusion
The electrolyte plays a crucial role in determining the performance, safety, and lifespan of lithium-ion batteries. This review has provided a comprehensive overview of the electrolyte landscape, encompassing its fundamental properties, composition, and interactions with electrode materials. While conventional liquid electrolytes have limitations in terms of flammability, electrochemical stability, and dendrite formation, emerging electrolyte chemistries and architectures, such as solid-state electrolytes, redox-active electrolytes, and localized high concentration electrolytes, offer promising solutions to these challenges. Continued research and development efforts are essential to unlocking the full potential of advanced electrolyte technologies and enabling the widespread adoption of high-performance, safe, and durable lithium-ion batteries for a sustainable energy future.
Many thanks to our sponsor Esdebe who helped us prepare this research report.
References
[1] Tarascon, J. M., & Armand, M. (2001). Issues and challenges facing rechargeable lithium batteries. Nature, 414(6861), 359-367.
[2] Xu, K. (2004). Nonaqueous liquid electrolytes for lithium-based rechargeable batteries. Chemical reviews, 104(10), 4303-4418.
[3] Zhang, S. S. (2006). A review on electrolyte additives for lithium-ion batteries. Journal of Power Sources, 162(2), 1379-1394.
[4] Goodenough, J. B., & Park, K. S. (2013). The Li-ion rechargeable battery: a perspective. Journal of the American Chemical Society, 135(4), 1167-1176.
[5] Newman, J., & Thomas-Alyea, K. E. (2012). Electrochemical systems. John Wiley & Sons.
[6] Doyle, M., Fuller, T. F., & Newman, J. (1993). Modeling of galvanostatic charge and discharge of the lithium/polymer/insertion cell. Journal of the Electrochemical Society, 140(6), 1526-1533.
[7] Bruce, P. G., Vincent, C. A., & Evans, I. M. (1987). Electrochemical behaviour of polymeric solid electrolytes. Polymer, 28(14), 2443-2448.
[8] Janek, J., & Zeier, W. G. (2016). A solid future for battery electrolytes?. Nature Energy, 1(9), 1-4.
[9] Borodin, O., Smith, G. D., & Douglas, J. F. (2003). Dynamics of electrolyte solutions for Li-ion batteries: a molecular dynamics simulation study. The Journal of Physical Chemistry B, 107(27), 6824-6832.
[10] Chen, S. R., Fan, L. Z., & Li, L. (2003). The effect of solvent composition on the electrochemical performance of LiNiO2 in liquid electrolytes. Journal of Power Sources, 115(2), 266-272.
[11] Valøen, L. O., & Reimers, J. N. (2005). Transport properties of LiPF6 solutions in ethylene carbonate, diethyl carbonate, and dimethyl carbonate. Journal of the Electrochemical Society, 152(5), A882-A891.
[12] Appetecchi, G. B., Carewska, M., Cassel, M. C., Croce, F., & Scrosati, B. (2000). Single lithium-ion conducting polymer electrolytes. Journal of the Electrochemical Society, 147(1), 57-63.
[13] Jung, R., Metzger, M., Haering, D., Stinner, C., Marino, C., Gasteiger, H. A., … & Lützenkirchen-Hecht, M. (2012). Aging mechanisms of Li-ion batteries in electric vehicles. Journal of The Electrochemical Society, 159(11), A1888-A1895.
[14] Balbuena, P. B., & Wang, Y. (2004). Lithium battery electrolytes. Solid State Ionics, 169(3-4), 173-179.
[15] Doughty, D. H., & Roth, E. P. (2012). A general discussion of Li ion battery safety. Electrochemical Society Interface, 21(2), 37-44.
[16] Manthiram, A. (2011). A vision for lithium-ion battery technology. Nature Communications, 2(1), 1-9.
[17] Lalia, B. S., Duluard, A., Bechelany, M., & Cretin, M. (2017). Recent advances in electrolytes for lithium-ion batteries. Journal of Power Sources, 368, 155-172.
[18] Xu, K. (2014). Electrolytes and interphases in Li-ion batteries and beyond. Chemical reviews, 114(23), 11503-11618.
[19] Croft, M., Fan, X., & Borodin, O. (2014). Perspective: Ion-pairing in LiPF6-based electrolytes. The Journal of Chemical Physics, 141(22), 220901.
[20] Yamada, Y., Yamada, A., Mukai, T., Ishikawa, T., & Abe, T. (2015). Highly concentrated organic liquid electrolytes for lithium-ion batteries. Chemical reviews, 115(23), 12985-13022.
[21] Petrat, F. M., Winter, M., & Kasnatscheew, J. (2016). Review on electrolyte oxidation and electrolyte additives for lithium-ion batteries with high-voltage positive electrodes. * Journal of Power Sources, 327, 44-64.
[22] Watanabe, M., Yamada, Y., Machida, T., Saito, T., Dokko, K., Nakashima, T., & Suga, T. (2012). Ionic liquids as electrolytes for lithium batteries. Chemical reviews, 112(6), 3405-3449.
[23] Janek, J., & Zeier, W. G. (2016). A solid future for battery electrolytes?. Nature Energy, 1(9), 1-4.
[24] Murugan, R., Weppner, W., & Maier, J. (2007). Lithium garnet-type solid electrolytes. Solid State Ionics, 178(19-21), 1465-1468.
[25] Subramanian, M. A., Uma, S., & Ramasamy, P. (2001). Lithium ion conductivity in the system Li3xLa (2/3)− xTiO3. Solid State Ionics, 136, 327-333.
[26] Fenton, D. E., Parker, J. M., & Wright, P. V. (1973). Complexes of alkali metal ions with poly (ethylene oxide). Polymer, 14(11), 589-589.
[27] Agrawal, R. C., & Gupta, G. P. (1999). Solid polymer electrolytes: materials designing and all-solid-state battery applications: an overview. Journal of Materials Science, 34(6), 1131-1160.
[28] Liu, W., Lu, Z., Zhao, J., Liu, G., Zhang, H., & Cui, Y. (2016). Redox-active organic compounds for high-capacity lithium-ion batteries. Energy & Environmental Science, 9(2), 453-473.
[29] Kundu, D., Black, R., & Nazar, L. F. (2015). Electrochemically tunable high-capacity organic cathode materials for rechargeable batteries. Advanced Energy Materials, 5(15), 1500373.
[30] Yamada, Y., Usui, K., Sodeyama, K., Watanabe, M., & Yamada, A. (2013). Unusual stability of highly concentrated ether electrolytes for fast-charging Li-ion batteries. Nature communications, 4(1), 1-7.
[31] Suo, L., Borodin, O., Gao, T., Olguin, M., Hoang, T., Fan, X., … & Wang, C. (2015). “Water-in-salt” electrolyte enables high-voltage lithium-ion chemistries. Science, 350(6263), 938-943.
[32] Peled, E. (1979). The electrochemical behavior of alkali and alkaline earth metals in nonaqueous electrolyte solutions—The solid electrolyte interphase concept. Journal of the Electrochemical Society, 126(12), 2047-2051.
[33] Verma, P., Maire, P., & Novák, P. (2010). A review of the self-discharge behaviour of lithium-ion batteries. Journal of Power Sources, 195(14), 4570-4585.
[34] Zheng, J., Gu, M., Chen, J., Meduri, P., Engelhard, M. H., Choi, D., … & Zhang, J. G. (2014). Understanding solid-electrolyte interphase of lithium metal anode. Accounts of chemical research, 47(8), 2657-2665.
[35] Kozen, A. C., Lin, C. H., Pearse, A. J., Vazquez-Arenas, J., Dahn, J. R., & Balsara, N. P. (2015). Suppression of lithium dendrite growth using hierarchical block copolymer electrolytes. Nature materials, 14(12), 1293-1301.
[36] Liu, W., Lee, S. H., & Manthiram, A. (2017). The effects of chemical and structural disorder on the electrochemical performance of high-nickel layered oxide cathodes for lithium-ion batteries. Journal of Materials Chemistry A, 5(25), 13123-13131.
[37] Jung, S. K., Gwon, H., Hong, J., Park, Y. C., & Choi, J. W. (2014). The effects of Al2O3 coating on LiNi0. 5Co0. 2Mn0. 3O2 cathode material for lithium-ion batteries. Journal of Power Sources, 246, 220-226.
[38] Chazalviel, J. N. (1990). Electrochemical aspects of the generation of dendritic deposits. Physical Review A, 42(12), 7355.
[39] Tikekar, M. D., Choudhury, S., Kojic, A., Mathew, K., Garcia, R. E., & Stevanovic, V. (2016). Designing solid-state electrolytes for lithium metal batteries: A combined computational and experimental approach. ACS applied materials & interfaces, 8(37), 24283-24292.
[40] Armand, M., Endres, F., MacFarlane, D. R., Ohno, H., & Scrosati, B. (2009). Ionic liquids—current developments and future trends. Energy & Environmental Science, 2(3), 267-278.
[41] Lewandowski, A., Świderska-Mocek, A. (2009). Ionic liquids as electrolytes for lithium ion batteries–an overview of electrochemical studies. Journal of Power Sources, 194(2), 561-569.
[42] Forsyth, M., & MacFarlane, D. R. (2003). Ionic conductivity of salt mixtures with the ionic liquid N-methyl-N-butylpyrrolidinium bis (trifluoromethylsulfonyl) imide. Journal of Physical Chemistry B, 107(36), 9501-9507.
[43] Abbott, A. P., Boothby, D., Capper, G., Davies, D. L., & Rasheed, R. K. (2004). Deep eutectic solvents formed between choline chloride and carboxylic acids: versatile alternatives to ionic liquids. Journal of the American Chemical Society, 126(29), 9142-9147.
[44] Fan, L. Z., Zhou, Y. L., Chen, S. R., Liu, X. J., Wang, G. C., & Tang, Y. (2006). Electrolytes based on room temperature ionic liquids for lithium ion batteries. Journal of Power Sources, 161(2), 1438-1442.
[45] Meyer, W. H. (1998). Polymer electrolytes for lithium rechargeable batteries. Advanced Materials, 10(6), 439-448.
[46] Croce, F., Appetecchi, G. B., Persi, L., & Scrosati, B. (2001). Nanocomposite polymer electrolytes for lithium batteries. Nature, 402(6763), 634-636.
[47] Zhang, S. S. (2006). A review on electrolyte additives for lithium-ion batteries. Journal of Power Sources, 162(2), 1379-1394.
[48] Ding, F., Xu, W., Graff, G. L., Zhang, J., Sushko, M. V., Chen, X., … & Zhang, J. G. (2013). Dendrite-free lithium deposition via self-regulated homogeneous Li+ flux. Journal of the American Chemical Society, 135(38), 14070-14073.
[49] Goodenough, J. B. (2007). The challenge of the solid electrolyte. Solid State Ionics, 178(19-21), 1449-1455.
[50] Li, W., Yao, H., Yan, K., Hasa, I., Zheng, L., Doeff, M. M., & Persson, K. A. (2020). High-nickel layered oxide cathode materials for lithium-ion batteries. Advanced Energy Materials, 10(48), 2000011.
[51] Yamada, Y., Yamada, A., Mukai, T., Ishikawa, T., & Abe, T. (2015). Highly concentrated organic liquid electrolytes for lithium-ion batteries. Chemical reviews, 115(23), 12985-13022.
[52] Christensen, J., Albertus, P., Austin, A., Davidson, A. R., Dunn, B., Mogensen, M., … & Viswanathan, V. (2012). Analysis of lithium-ion battery degradation mechanisms using electrochemical impedance spectroscopy. Journal of The Electrochemical Society, 159*(2), A193-A199.
This is a great overview of the challenges in electrolyte development. The discussion of localized high concentration electrolytes (LHCEs) is particularly interesting. Could you elaborate on how the choice of diluent in LHCEs impacts the formation and stability of the SEI layer?
Thanks for your insightful comment! The choice of diluent in LHCEs significantly influences SEI formation by modulating the solvation structure and electrochemical stability of the electrolyte. A carefully selected diluent can promote the formation of a robust and ionically conductive SEI, leading to improved battery performance and cycle life. Let’s discuss further!
Editor: MedTechNews.Uk
Thank you to our Sponsor Esdebe
That’s quite the deep dive! Given the issues with dendrite formation, could “structured” electrolytes become a key strategy? Perhaps 3D-printed scaffolds to guide ion flow, ensuring even deposition? Just imagine the possibilities!
Thanks for your comment! That’s a really interesting point about structured electrolytes. 3D-printed scaffolds could indeed offer a pathway to control ion flow. This precise control might also allow for the use of novel electrolyte compositions that are currently unstable due to dendrite formation.
Editor: MedTechNews.Uk
Thank you to our Sponsor Esdebe