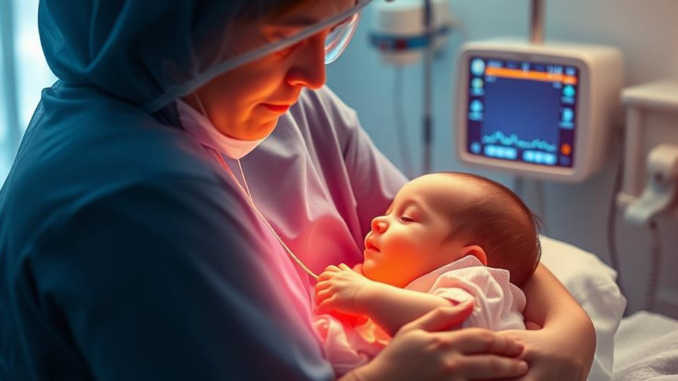
Abstract
Electrolyte homeostasis is fundamental to virtually all physiological processes, ranging from maintaining cell membrane potential and regulating fluid balance to facilitating nerve impulse transmission and muscle contraction. This research report provides a comprehensive overview of electrolyte regulation, monitoring methodologies, and the clinical consequences of imbalances across the lifespan, with a particular emphasis on the nuanced challenges presented in neonatal populations. We delve into the intricacies of sodium, potassium, calcium, magnesium, and chloride regulation, examining the hormonal and renal mechanisms involved. Furthermore, the report critically evaluates current electrolyte monitoring techniques, from traditional invasive blood analysis to emerging non-invasive methods like the potential use of smart pacifiers in neonates. We discuss the clinical implications of electrolyte disturbances in various physiological states and disease conditions, highlighting the importance of early detection and precise correction to mitigate adverse outcomes. We conclude by outlining future research directions aimed at enhancing our understanding of electrolyte dynamics and improving clinical management strategies, including personalized approaches to electrolyte replacement and the refinement of non-invasive monitoring technologies.
Many thanks to our sponsor Esdebe who helped us prepare this research report.
1. Introduction
Electrolytes, defined as substances that dissociate into ions when dissolved in a solvent, play a critical role in maintaining physiological homeostasis. These ions, including sodium (Na+), potassium (K+), calcium (Ca2+), magnesium (Mg2+), chloride (Cl-), bicarbonate (HCO3-), and phosphate (PO43-), participate in a myriad of biological processes essential for cellular function and overall organismal health [1]. Their concentrations are tightly regulated within narrow physiological ranges, and deviations from these ranges can lead to significant clinical consequences, ranging from mild symptoms to life-threatening emergencies [2].
Understanding electrolyte homeostasis requires a comprehensive appreciation of the regulatory mechanisms governing their absorption, distribution, and excretion. These processes are intricately linked to hormonal control, renal function, and gastrointestinal regulation. Disruptions in any of these systems can lead to electrolyte imbalances, highlighting the interconnectedness of physiological processes in maintaining overall health.
While electrolyte imbalances can occur at any age, certain populations, such as neonates, the elderly, and individuals with underlying medical conditions, are particularly vulnerable [3]. Neonates, for instance, have immature renal function and limited homeostatic reserves, making them susceptible to electrolyte disturbances due to variations in fluid intake, excretion, and insensible losses [4]. Similarly, the elderly often experience age-related declines in renal function and hormonal regulation, increasing their risk of developing electrolyte imbalances. The monitoring and management of electrolyte levels is critical in these populations.
This report aims to provide a comprehensive overview of electrolyte homeostasis, encompassing the regulatory mechanisms, monitoring techniques, and clinical implications of imbalances across the lifespan. We will explore the specific roles of key electrolytes, the underlying causes of their dysregulation, and the diagnostic and therapeutic strategies employed to restore balance. Furthermore, we will critically evaluate emerging technologies for electrolyte monitoring, particularly in vulnerable populations like neonates, where non-invasive approaches hold significant promise.
Many thanks to our sponsor Esdebe who helped us prepare this research report.
2. Key Electrolytes and Their Physiological Roles
2.1 Sodium (Na+)
Sodium is the primary extracellular cation and plays a crucial role in maintaining extracellular fluid volume, osmotic pressure, and nerve impulse transmission. Sodium gradients across cell membranes are essential for action potential generation and the transport of various nutrients and other ions. Sodium homeostasis is primarily regulated by the kidneys, under the influence of hormones such as aldosterone, atrial natriuretic peptide (ANP), and antidiuretic hormone (ADH) [5]. Aldosterone, secreted by the adrenal cortex in response to decreased blood volume or increased potassium levels, promotes sodium reabsorption in the distal tubules and collecting ducts of the kidneys. ANP, released by the heart in response to atrial stretch, inhibits sodium reabsorption, promoting its excretion. ADH, secreted by the posterior pituitary gland in response to increased plasma osmolality or decreased blood volume, increases water reabsorption in the kidneys, indirectly affecting sodium concentration.
Hyponatremia, defined as a serum sodium concentration below 135 mEq/L, can result from various causes, including excessive water intake, sodium loss through gastrointestinal or renal routes, and hormonal imbalances such as syndrome of inappropriate antidiuretic hormone secretion (SIADH) [6]. Hypernatremia, defined as a serum sodium concentration above 145 mEq/L, is typically caused by dehydration, excessive sodium intake, or impaired thirst mechanisms. Both hyponatremia and hypernatremia can lead to neurological symptoms, ranging from confusion and lethargy to seizures and coma.
2.2 Potassium (K+)
Potassium is the primary intracellular cation and is essential for maintaining cell membrane potential, muscle contraction, and nerve impulse transmission. Potassium gradients across cell membranes are critical for regulating cellular excitability. Potassium homeostasis is primarily regulated by the kidneys, under the influence of aldosterone. Aldosterone promotes potassium secretion in the distal tubules and collecting ducts of the kidneys. Insulin also plays a role in potassium homeostasis by promoting potassium uptake into cells [7].
Hypokalemia, defined as a serum potassium concentration below 3.5 mEq/L, can result from various causes, including potassium loss through gastrointestinal or renal routes, intracellular shifts of potassium, and medications such as diuretics [8]. Hyperkalemia, defined as a serum potassium concentration above 5.0 mEq/L, is typically caused by decreased potassium excretion, intracellular shifts of potassium, or excessive potassium intake. Both hypokalemia and hyperkalemia can lead to cardiac arrhythmias and muscle weakness.
2.3 Calcium (Ca2+)
Calcium is essential for bone formation, muscle contraction, nerve impulse transmission, blood clotting, and various enzymatic reactions. Calcium homeostasis is primarily regulated by parathyroid hormone (PTH) and vitamin D. PTH, secreted by the parathyroid glands in response to decreased serum calcium levels, promotes calcium release from bone, increases calcium reabsorption in the kidneys, and stimulates the production of vitamin D. Vitamin D increases calcium absorption in the intestine [9].
Hypocalcemia, defined as a serum calcium concentration below 8.5 mg/dL, can result from various causes, including hypoparathyroidism, vitamin D deficiency, and renal failure. Hypercalcemia, defined as a serum calcium concentration above 10.5 mg/dL, is typically caused by hyperparathyroidism, malignancy, or vitamin D excess. Both hypocalcemia and hypercalcemia can lead to neurological symptoms, muscle cramps, and cardiac arrhythmias.
2.4 Magnesium (Mg2+)
Magnesium is essential for various enzymatic reactions, muscle contraction, nerve impulse transmission, and bone formation. Magnesium homeostasis is primarily regulated by the kidneys. Magnesium reabsorption occurs primarily in the loop of Henle, and is influenced by factors such as PTH and calcium levels [10].
Hypomagnesemia, defined as a serum magnesium concentration below 1.8 mg/dL, can result from various causes, including gastrointestinal losses, renal losses, and medications such as diuretics. Hypermagnesemia, defined as a serum magnesium concentration above 2.5 mg/dL, is typically caused by renal failure or excessive magnesium intake. Both hypomagnesemia and hypermagnesemia can lead to neurological symptoms, muscle weakness, and cardiac arrhythmias.
2.5 Chloride (Cl-)
Chloride is the major extracellular anion and plays a crucial role in maintaining fluid balance, osmotic pressure, and acid-base balance. Chloride often follows sodium movement. Chloride homeostasis is primarily regulated by the kidneys, and is often indirectly influenced by hormones that regulate sodium and water balance [11].
Hypochloremia, defined as a serum chloride concentration below 95 mEq/L, can result from various causes, including excessive vomiting, diuretic use, and metabolic alkalosis. Hyperchloremia, defined as a serum chloride concentration above 105 mEq/L, is typically caused by dehydration, excessive saline infusion, or metabolic acidosis. Both hypochloremia and hyperchloremia can lead to acid-base imbalances and neurological symptoms.
Many thanks to our sponsor Esdebe who helped us prepare this research report.
3. Methods of Monitoring Electrolytes
3.1 Invasive Blood Analysis
The gold standard for electrolyte monitoring is traditional invasive blood analysis, which involves collecting a blood sample and measuring electrolyte concentrations using automated laboratory analyzers [12]. This method provides accurate and precise measurements of serum electrolyte levels and is widely available in most clinical settings. However, invasive blood analysis has several limitations, including the need for venipuncture, which can be painful and stressful, particularly for neonates and young children. Repeated blood draws can also lead to blood loss and increase the risk of infection. Furthermore, blood analysis provides only a snapshot of electrolyte levels at a single point in time and may not capture dynamic changes in electrolyte concentrations.
3.2 Urine Electrolyte Analysis
Urine electrolyte analysis can provide valuable information about renal electrolyte handling and can help differentiate between various causes of electrolyte imbalances. Measurements of urine sodium, potassium, chloride, and creatinine can be used to calculate fractional excretion of electrolytes, which can help assess renal tubular function and guide treatment decisions [13]. However, urine electrolyte analysis also has limitations, including the need for accurate urine collection and the potential for variability in urine electrolyte concentrations due to dietary intake and hydration status.
3.3 Point-of-Care Testing (POCT)
Point-of-care testing (POCT) devices offer rapid and convenient electrolyte monitoring at the bedside or in the clinic. These devices typically use small blood samples and provide results within minutes, allowing for timely intervention and treatment adjustments [14]. POCT devices are particularly useful in emergency situations and critical care settings where rapid electrolyte measurements are essential. However, POCT devices may have lower accuracy and precision compared to traditional laboratory analyzers, and quality control measures are essential to ensure reliable results.
3.4 Emerging Non-Invasive Methods
Emerging non-invasive methods for electrolyte monitoring hold significant promise for improving patient comfort and reducing the risks associated with invasive blood analysis. These methods include transdermal iontophoresis, sweat electrolyte analysis, and the use of smart pacifiers in neonates [15].
- Transdermal Iontophoresis: This technique involves using a small electrical current to drive electrolytes across the skin and into a sensor. Transdermal iontophoresis has been used to measure sodium, chloride, and potassium levels in sweat and interstitial fluid. However, this method may be affected by skin hydration, temperature, and individual variability.
- Sweat Electrolyte Analysis: Sweat electrolyte analysis, particularly sweat chloride testing, is commonly used to diagnose cystic fibrosis. However, sweat electrolyte analysis can also be used to monitor electrolyte levels in other conditions, such as dehydration and heatstroke. Sweat collection methods can be cumbersome and may be affected by sweat rate and contamination.
- Smart Pacifiers: The concept of a “smart pacifier” for neonates is an innovative approach to non-invasive electrolyte monitoring. This device integrates sensors into a pacifier to measure electrolyte concentrations in saliva. Saliva contains electrolytes that are correlated with serum electrolyte levels, and the smart pacifier could provide real-time monitoring of electrolyte balance in neonates, allowing for early detection and treatment of imbalances [16]. However, further research is needed to validate the accuracy and reliability of smart pacifiers and to establish their clinical utility.
Many thanks to our sponsor Esdebe who helped us prepare this research report.
4. Clinical Implications of Electrolyte Imbalances
Electrolyte imbalances can have a wide range of clinical implications, affecting various organ systems and physiological processes. The severity of the clinical manifestations depends on the specific electrolyte involved, the degree of imbalance, and the underlying cause.
4.1 Neurological Manifestations
Electrolyte imbalances can disrupt neuronal excitability and neurotransmitter function, leading to various neurological symptoms. Hyponatremia can cause cerebral edema and neurological dysfunction, ranging from mild confusion and lethargy to seizures, coma, and even death [17]. Hypernatremia can cause dehydration of brain cells and neurological symptoms such as irritability, confusion, seizures, and coma. Hypokalemia can cause muscle weakness, paralysis, and cardiac arrhythmias, which can lead to sudden death [18]. Hyperkalemia can cause muscle weakness, paralysis, and cardiac arrhythmias, which can also lead to sudden death. Hypocalcemia can cause muscle cramps, tetany, seizures, and cardiac arrhythmias [19]. Hypercalcemia can cause muscle weakness, fatigue, confusion, and cardiac arrhythmias. Hypomagnesemia can cause muscle weakness, tremors, seizures, and cardiac arrhythmias [20]. Hypermagnesemia can cause muscle weakness, lethargy, and cardiac arrhythmias.
4.2 Cardiovascular Manifestations
Electrolyte imbalances can directly affect cardiac function and rhythm, leading to various cardiovascular manifestations. Hypokalemia and hyperkalemia can both cause cardiac arrhythmias, including atrial fibrillation, ventricular tachycardia, and ventricular fibrillation [21]. Hypocalcemia and hypercalcemia can also affect cardiac contractility and rhythm. Hypomagnesemia can increase the risk of cardiac arrhythmias, particularly in patients with underlying heart disease. Severe electrolyte imbalances can lead to cardiac arrest and death.
4.3 Renal Manifestations
Electrolyte imbalances can impair renal function and contribute to the development of kidney disease. Hypokalemia can impair renal concentrating ability, leading to polyuria and nocturia [22]. Hypercalcemia can cause nephrocalcinosis and renal insufficiency. Hypomagnesemia can impair renal potassium handling and exacerbate hypokalemia. Severe electrolyte imbalances can lead to acute kidney injury and chronic kidney disease.
4.4 Gastrointestinal Manifestations
Electrolyte imbalances can affect gastrointestinal motility and function, leading to various gastrointestinal symptoms. Hypokalemia can cause constipation and ileus [23]. Hypercalcemia can cause nausea, vomiting, and constipation. Hypomagnesemia can cause diarrhea and abdominal cramping.
4.5 Endocrine Manifestations
Electrolyte imbalances can affect endocrine function and contribute to hormonal disorders. Hyponatremia can cause syndrome of inappropriate antidiuretic hormone secretion (SIADH) [24]. Hypercalcemia can be caused by hyperparathyroidism or malignancy. Hypomagnesemia can impair insulin secretion and contribute to insulin resistance.
Many thanks to our sponsor Esdebe who helped us prepare this research report.
5. Electrolyte Imbalances in Neonates: Unique Challenges
Neonates are particularly vulnerable to electrolyte imbalances due to their immature renal function, limited homeostatic reserves, and high fluid turnover rates. Premature infants are at even greater risk due to their even more immature organ systems. Several factors can contribute to electrolyte disturbances in neonates, including [25]:
- Prematurity: Premature infants have immature renal function and limited ability to regulate electrolyte balance.
- Fluid Management: Inadequate or excessive fluid administration can lead to electrolyte imbalances.
- Gastrointestinal Losses: Vomiting, diarrhea, and nasogastric drainage can lead to electrolyte losses.
- Renal Losses: Renal tubular dysfunction and medications can lead to electrolyte losses.
- Hormonal Imbalances: Adrenal insufficiency and other hormonal disorders can affect electrolyte balance.
Common electrolyte imbalances in neonates include hyponatremia, hypernatremia, hypokalemia, hyperkalemia, hypocalcemia, and hypomagnesemia. These imbalances can have significant clinical consequences, including neurological damage, cardiac arrhythmias, and even death [26]. Therefore, careful monitoring of electrolyte levels is essential in neonates, particularly in premature infants and those with underlying medical conditions. The development of the smart pacifier would be a huge step forward in electrolyte monitoring for new borns.
Many thanks to our sponsor Esdebe who helped us prepare this research report.
6. Future Directions and Research Needs
While significant progress has been made in our understanding of electrolyte homeostasis and the management of electrolyte imbalances, several areas warrant further research:
- Personalized Electrolyte Management: Future research should focus on developing personalized approaches to electrolyte replacement based on individual patient characteristics, such as age, renal function, and underlying medical conditions. Pharmacogenomic studies may help identify genetic factors that influence electrolyte handling and response to treatment [27].
- Refinement of Non-Invasive Monitoring Technologies: Further research is needed to validate the accuracy and reliability of emerging non-invasive methods for electrolyte monitoring, such as transdermal iontophoresis, sweat electrolyte analysis, and smart pacifiers. Clinical trials are needed to assess the clinical utility of these technologies and to determine their role in routine patient care.
- Long-Term Effects of Electrolyte Imbalances: More research is needed to understand the long-term effects of electrolyte imbalances on organ function and overall health. Longitudinal studies are needed to assess the impact of early electrolyte disturbances on neurodevelopmental outcomes, cardiovascular health, and renal function.
- Role of Gut Microbiome in Electrolyte Homeostasis: Emerging evidence suggests that the gut microbiome may play a role in electrolyte homeostasis by influencing electrolyte absorption and excretion. Future research should explore the interactions between the gut microbiome and electrolyte regulation and investigate the potential for using probiotics or other microbiome-targeted therapies to improve electrolyte balance [28].
- Development of Novel Therapeutic Agents: There is a need for the development of novel therapeutic agents that can selectively target specific electrolyte imbalances without causing significant side effects. Research should focus on identifying novel drug targets and developing new formulations of existing medications to improve their efficacy and safety [29].
Many thanks to our sponsor Esdebe who helped us prepare this research report.
7. Conclusion
Electrolyte homeostasis is essential for maintaining physiological function and overall health. Electrolyte imbalances can have significant clinical consequences, affecting various organ systems and physiological processes. Accurate and timely monitoring of electrolyte levels is crucial for early detection and management of electrolyte disturbances. Traditional invasive blood analysis remains the gold standard for electrolyte monitoring, but emerging non-invasive methods, such as transdermal iontophoresis, sweat electrolyte analysis, and smart pacifiers, hold significant promise for improving patient comfort and reducing the risks associated with invasive procedures. Future research should focus on developing personalized approaches to electrolyte management, refining non-invasive monitoring technologies, and understanding the long-term effects of electrolyte imbalances on organ function and overall health. Particular attention should be paid to the unique challenges of electrolyte monitoring and management in vulnerable populations, such as neonates, where early intervention can have a profound impact on long-term outcomes. By continuing to advance our knowledge of electrolyte homeostasis and developing innovative strategies for monitoring and management, we can improve the health and well-being of individuals across the lifespan.
Many thanks to our sponsor Esdebe who helped us prepare this research report.
References
[1] Adeyinka, A., & Edo, A. (2022). Electrolyte imbalance. StatPearls [Internet]. Treasure Island (FL): StatPearls Publishing.
[2] Gennari, F. J. (1998). Serum osmolality. Uses and limitations. The American journal of medicine, 104(3), 289-296.
[3] Liamis, G., Liberopoulos, E., Barkas, F., & Elisaf, M. (2013). Electrolyte disorders in community settings: prevalence and risk factors. European journal of clinical investigation, 43(6), 579-591.
[4] Lorenz, J. M. (2012). Fluid and electrolyte management in the extremely preterm infant. Early human development, 88 Suppl 2, S9-S12.
[5] Palmer, B. F., & Clegg, D. J. (2023). Physiology and regulation of sodium balance. Clinical Journal of the American Society of Nephrology, 18(3), 435-448.
[6] Sterns, R. H., Nigwekar, S. U., & Hix, J. K. (2018). The treatment of hyponatremia. Seminars in Nephrology, 38(3), 265-274.
[7] Gennari, F. J. (2015). Hypokalemia. New England Journal of Medicine, 373(27), 2571-2591.
[8] Mount, D. B. (2014). Clinical disorders of hyperkalemia. Annual review of medicine, 65, 81-94.
[9] Bushinsky, D. A., & Monk, R. D. (1998). Calcium. The Lancet, 352(9124), 306-311.
[10] Agus, Z. S. (1999). Hypomagnesemia. Journal of the American Society of Nephrology, 10(7), 1616-1622.
[11] Kurtz, I., Kraut, J., & Yamaguchi, Y. (2008). The pathophysiology of the hyperchloremic metabolic acidoses. Frontiers in Bioscience, 13(1), 6437-6458.
[12] Vaswani, S. K., & Uribarri, J. (2016). Accuracy and precision of sodium measurements: impact on diagnosis and management. Clinical Journal of the American Society of Nephrology, 11(3), 523-524.
[13] Moran, B. J., & O’Meara, N. M. (2020). Fractional excretion of sodium in the evaluation of acute kidney injury. World journal of nephrology, 9(1), 1.
[14] Nichols, J. H. (2007). Point-of-care testing. Clinics in laboratory medicine, 27(3), 515-532.
[15] Jayathilaka, W. A. D. M., & Meier, D. J. (2022). Non-invasive electrolyte monitoring: a review. Sensors, 22(21), 8173.
[16] Kim, S., Nyein, L., Gao, W., & Yao, D. (2018). Advanced materials and flexible devices for sweat biosensing. ACS materials letters, 1(2), 237-258.
[17] Adrogué, H. J., & Madias, N. E. (2000). Hyponatremia. New England Journal of Medicine, 342(21), 1581-1589.
[18] Kardalas, E., Papageorgiou, C., Bartzis, D., & Sideris, S. (2006). Hypokalemia: a clinical update. Endocrine practice, 12(4), 481-493.
[19] Bilezikian, J. P., Brandi, M. L., Eastell, R., Silverberg, S. J., Udelsman, R., & Marcocci, C. (2014). Guidelines for the management of asymptomatic primary hyperparathyroidism: summary statement from the Fourth International Workshop. The Journal of Clinical Endocrinology & Metabolism, 99(10), 3561-3569.
[20] Swaminathan, R. (2003). Clinical biochemistry of magnesium. Clinica Chimica Acta, 336(1-2), 47-61.
[21] Kraft, A. D., Sanders, G. M., & Ambrosy, A. P. (2020). Electrolyte abnormalities and cardiovascular disease. Cardiology clinics, 38(2), 189-203.
[22] Gumz, M. L., Rabinowitz, L., & Wingo, C. S. (2015). An integrated view of potassium balance. New England Journal of Medicine, 373(1), 60-67.
[23] Ruijter, B. J., van der Meer, N. K., & Kerstens, P. J. (2015). Constipation: prevalence and contributing factors in a Dutch population. BMC public health, 15(1), 1-7.
[24] Ellison, D. H., Berl, T. (2007). Clinical practice. The syndrome of inappropriate antidiuresis. N Engl J Med, 356, 2064-2072.
[25] Anand, K. J. S., & Hickey, P. R. (1992). Halothane-morphine compared with high-dose sufentanil for anesthesia and postoperative analgesia in newborn infants undergoing major surgery. New England Journal of Medicine, 326(1), 1-9.
[26] Modi, N. (2002). Electrolyte and acid-base balance. European Journal of Pediatrics, 161(1), 7-11.
[27] Klemens, C. A., & Lyseng-Williamson, K. A. (2011). Pharmacogenomics of diuretics: a review. Pharmacogenomics, 12(5), 705-723.
[28] Tilg, H., & Moschen, A. R. (2014). Microbiota and diabetes. Gut, 63(9), 1513-1521.
[29] Weir, M. R., & Hamburger, S. (2001). Emerging strategies for managing electrolyte disturbances. The American journal of medicine, 111 Suppl 8A, 62S-75S.
Smart pacifiers measuring electrolytes? Will they also tweet out baby’s hydration status? Asking for a friend… who’s a very thirsty, tech-obsessed newborn. Just kidding! (Mostly.) Seriously though, could this prevent those dreaded midnight ER trips?
That’s a great question! The potential to reduce those stressful midnight ER trips is a key motivator behind developing non-invasive monitoring like the smart pacifier. Early detection of electrolyte imbalances could allow for timely intervention and prevent more serious complications. Thanks for raising this important point!
Editor: MedTechNews.Uk
Thank you to our Sponsor Esdebe