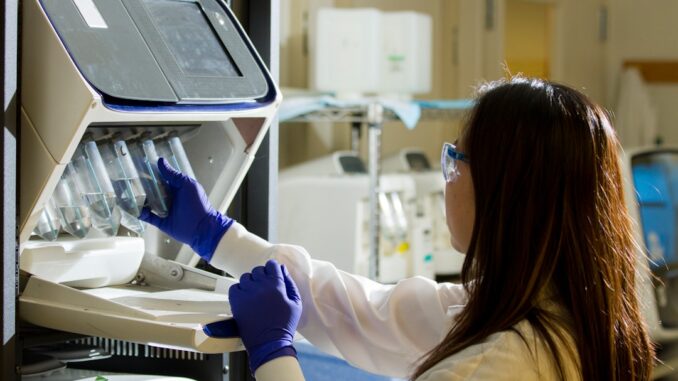
Abstract
Electroporation, the phenomenon of increasing cell membrane permeability through the application of external electric fields, has evolved from a fundamental biophysical observation to a versatile tool with broad applications in biotechnology and medicine. This report delves into the intricate mechanisms of action underlying electroporation, focusing on the dynamic changes induced in cell membranes, the formation and evolution of pores, and the influence of pulse parameters. Furthermore, the report explores the potential of electroporation for targeted drug and gene delivery, highlighting the strategies employed to enhance selectivity and efficacy. Beyond its applications in diabetes treatment (e.g., ReCET therapy), the report comprehensively examines the long-term safety and efficacy of electroporation across a diverse range of medical applications, including cancer therapy, gene therapy, and transdermal drug delivery. The review integrates current understanding of the biophysical principles with clinical outcomes, aiming to provide a comprehensive overview of the current state and future prospects of electroporation technology.
Many thanks to our sponsor Esdebe who helped us prepare this research report.
1. Introduction
Electroporation, also known as electropermeabilization, is a technique that employs brief, intense electrical pulses to transiently destabilize the cell membrane, thereby increasing its permeability to molecules that would normally be impermeable. This phenomenon has found widespread use in various scientific and medical fields, ranging from fundamental research in cell biology and genetics to advanced therapies such as drug and gene delivery, cancer treatment, and regenerative medicine. The efficacy of electroporation depends critically on several parameters, including the electric field strength, pulse duration, pulse number, pulse waveform, and the specific characteristics of the target cells or tissues. While the immediate effects of electroporation, such as increased membrane permeability and facilitated molecular transport, are well-documented, the long-term consequences on cellular function, tissue architecture, and overall patient health require thorough investigation.
The initial observation of electroporation dates back to the 1950s, when researchers noted the reversible breakdown of cell membranes under strong electric fields. However, it was not until the 1980s that the technique gained widespread recognition and practical application, driven by advancements in pulse generator technology and a growing understanding of the underlying biophysical mechanisms. Early applications primarily focused on the introduction of DNA into cells for genetic engineering purposes. Over time, the versatility of electroporation has expanded significantly, leading to its adoption in diverse fields.
The recent interest in electroporation within the context of ReCET therapy for diabetes has spurred renewed investigation into its potential, but this report will focus on the broader spectrum of applications and address critical aspects, including membrane dynamics, targeted delivery strategies, and long-term safety profiles across various therapeutic modalities.
Many thanks to our sponsor Esdebe who helped us prepare this research report.
2. Mechanisms of Action: A Biophysical Perspective
The precise mechanisms governing electroporation are complex and involve a cascade of events at the molecular and cellular level. The application of an external electric field across a cell membrane induces a transmembrane potential (TMP), which is the voltage difference between the inside and outside of the cell. The induced TMP is proportional to the radius of the cell and the electric field strength. When the induced TMP exceeds a critical threshold (typically around 0.5-1 V), the cell membrane undergoes destabilization, leading to the formation of aqueous pores. These pores are not simply “holes” but rather dynamic structures composed of rearranged lipid molecules and associated proteins.
2.1. Pore Formation and Stability
The prevailing theory suggests that pore formation initiates with the reorientation and clustering of polar headgroups of lipid molecules in the cell membrane. These clusters create areas of increased hydrophilicity, attracting water molecules and initiating the formation of water-filled defects. As the electric field is sustained, these defects expand and coalesce, eventually forming stable pores. The lifetime and size distribution of these pores are influenced by several factors, including the electric field parameters, lipid composition of the membrane, and temperature.
Recent research has focused on characterizing the dynamics of pore formation and closure using advanced imaging techniques such as molecular dynamics simulations and high-speed microscopy. These studies have revealed that pores can exhibit a range of sizes, from nanometers to micrometers, and that their stability is governed by a delicate balance between electrostatic forces, hydrophobic interactions, and membrane tension. Furthermore, the presence of membrane proteins, such as ion channels and receptors, can influence pore formation and stability by acting as nucleation sites or by altering the local lipid environment.
2.2. Influence of Pulse Parameters
The effectiveness of electroporation is highly sensitive to the electric pulse parameters. The electric field strength determines the magnitude of the induced TMP, while the pulse duration dictates the time available for pore formation and molecular transport. Short, high-intensity pulses typically result in transient pore formation, suitable for delivering small molecules, whereas longer, lower-intensity pulses can induce more sustained pore formation, facilitating the entry of larger macromolecules such as DNA. The pulse number and pulse waveform also play crucial roles in determining the overall outcome of electroporation. Multiple pulses can enhance molecular transport by increasing the cumulative pore area and extending the permeabilization time. Different pulse waveforms, such as square wave, exponential decay, and oscillating pulses, can exhibit distinct effects on cell viability and transfection efficiency. For example, some evidence suggests that bipolar pulses are more effective at minimizing cell damage compared to monopolar pulses. It’s important to note that there are conflicting opinions in the field about the optimal waveform for particular applications, highlighting the complexity of the interaction between pulse parameters and cellular response.
2.3. Cell Type Specificity
The susceptibility of cells to electroporation varies depending on cell type, size, morphology, and membrane composition. Larger cells typically exhibit a higher induced TMP and are more readily permeabilized than smaller cells. Cells with irregular shapes or complex membrane structures may also exhibit variations in pore formation and distribution. The lipid composition of the cell membrane, particularly the ratio of cholesterol to phospholipids and the presence of specific lipid domains (e.g., lipid rafts), can influence membrane fluidity and stability, thereby affecting the response to electroporation. Understanding these cell type-specific differences is essential for optimizing electroporation protocols for specific applications and minimizing off-target effects.
Many thanks to our sponsor Esdebe who helped us prepare this research report.
3. Effects on Cell Membranes: Structure, Function, and Repair
Electroporation induces a variety of structural and functional changes in cell membranes beyond simply pore formation. These changes can affect membrane integrity, fluidity, permeability, and interactions with the extracellular environment. The extent and reversibility of these changes depend on the electroporation parameters and the intrinsic properties of the cell membrane.
3.1. Membrane Lipid Reorganization
Electroporation can induce significant changes in the lateral organization of membrane lipids. The formation of pores disrupts the normal lipid packing, leading to increased membrane fluidity and the redistribution of lipid domains. Cholesterol, a key regulator of membrane fluidity, can be displaced from its normal position within the lipid bilayer, affecting the stability and permeability of the membrane. Furthermore, electroporation can induce the formation of non-bilayer lipid structures, such as inverted micelles, which can further destabilize the membrane and facilitate the transport of molecules across the membrane.
3.2. Protein Interactions and Translocation
Electroporation can also affect the distribution and function of membrane proteins. The induced electric field can exert forces on charged protein domains, leading to protein conformational changes and lateral movement within the membrane. Furthermore, the increased membrane permeability can facilitate the translocation of proteins across the membrane, potentially disrupting cellular signaling pathways and transport processes. Certain membrane proteins, such as ion channels and receptors, may be directly affected by the electric field, leading to altered channel gating or receptor activation. This can have significant consequences for cellular excitability, ion homeostasis, and cellular communication. There is evidence that electroporation can transiently increase the activity of certain ion channels, which can then lead to downstream effects on cell signaling.
3.3. Membrane Repair Mechanisms
Cells possess intrinsic membrane repair mechanisms that are activated in response to electroporation-induced damage. These mechanisms involve the recruitment of membrane repair proteins, such as annexins and ESCRT proteins, to the site of damage, followed by the resealing of the membrane. The efficiency of membrane repair depends on the severity of the damage and the availability of cellular resources. In some cases, cells may be unable to repair the damage, leading to cell death via apoptosis or necrosis. Understanding the cellular mechanisms involved in membrane repair is crucial for optimizing electroporation protocols and minimizing cell damage.
3.4. Long-Term Consequences on Membrane Function
While electroporation is often considered a transient and reversible process, the long-term consequences on membrane function are not fully understood. Repeated or prolonged exposure to electric fields can induce cumulative damage to the membrane, leading to chronic alterations in membrane permeability, lipid composition, and protein distribution. These changes can affect cellular function, viability, and response to external stimuli. Further research is needed to fully elucidate the long-term effects of electroporation on membrane function and to develop strategies to mitigate any potential adverse consequences.
Many thanks to our sponsor Esdebe who helped us prepare this research report.
4. Electroporation for Targeted Drug and Gene Delivery
The ability to deliver therapeutic molecules specifically to target cells or tissues is a major goal in drug and gene therapy. Electroporation offers a promising approach for achieving targeted delivery by selectively permeabilizing the membranes of target cells while sparing non-target cells. Several strategies have been developed to enhance the selectivity and efficacy of electroporation for targeted delivery.
4.1. Localized Electroporation
One approach for achieving targeted delivery is to restrict the electric field to a specific region of the body. This can be accomplished by using specialized electrode configurations or by applying the electric field directly to the target tissue. Localized electroporation minimizes off-target effects and reduces the systemic exposure to therapeutic molecules. For example, in cancer therapy, localized electroporation can be used to deliver chemotherapeutic drugs directly to the tumor, sparing surrounding healthy tissues. This approach can significantly improve the therapeutic index of anticancer drugs and reduce the severity of side effects.
4.2. Cell-Specific Electroporation
Another strategy for targeted delivery is to exploit the differences in electrical properties or membrane characteristics between target and non-target cells. Cells with higher membrane capacitance or larger size are more susceptible to electroporation than smaller cells with lower capacitance. By carefully adjusting the electric field parameters, it is possible to selectively permeabilize the membranes of target cells while leaving non-target cells relatively unaffected. Furthermore, cells can be pre-treated with agents that sensitize them to electroporation, further enhancing the selectivity of the process. These agents may include chemicals that alter membrane fluidity, disrupt the cytoskeleton, or modulate the expression of membrane proteins.
4.3. Electroporation-Enhanced Antibody Delivery
Antibodies that bind specifically to target cells can be used to guide therapeutic molecules to the desired location. By conjugating therapeutic molecules to antibodies or by co-administering antibodies with therapeutic molecules, it is possible to achieve targeted delivery via receptor-mediated endocytosis. Electroporation can enhance the uptake of antibody-drug conjugates or antibody-therapeutic complexes by increasing the membrane permeability and facilitating the transport of these molecules across the cell membrane. This approach can be particularly effective for delivering therapeutic molecules to intracellular targets that are inaccessible by conventional methods.
4.4. Gene Electrotransfer
Gene electrotransfer (GET), also known as electroporation-mediated gene delivery, is a widely used technique for introducing genetic material into cells. GET involves the administration of DNA or RNA into the target tissue, followed by the application of electric pulses to facilitate the entry of the genetic material into the cells. GET has been successfully used in a variety of applications, including gene therapy, DNA vaccination, and gene editing. The efficiency of GET is influenced by several factors, including the type of genetic material, the electric field parameters, and the target tissue. In recent years, significant progress has been made in improving the efficiency and safety of GET. This includes the development of novel electrode designs, optimized pulse protocols, and improved formulations of genetic material. One of the main challenges in GET is to achieve long-term expression of the delivered genes. This can be addressed by using viral vectors or by incorporating the delivered genes into the host cell genome.
Many thanks to our sponsor Esdebe who helped us prepare this research report.
5. Long-Term Safety and Efficacy in Medical Applications
The safety and efficacy of electroporation are critical considerations for its widespread adoption in medical applications. While electroporation is generally considered to be a safe and well-tolerated procedure, potential risks and side effects need to be carefully evaluated. The long-term consequences of electroporation on tissue architecture, cellular function, and overall patient health require thorough investigation. The following applications serve as examples:
5.1. Cancer Therapy
Electrochemotherapy (ECT), a combination of electroporation and chemotherapy, has emerged as a promising treatment for various types of cancer. ECT involves the administration of a chemotherapeutic drug, such as bleomycin or cisplatin, followed by the application of electric pulses to the tumor. Electroporation enhances the uptake of the chemotherapeutic drug by the tumor cells, resulting in increased cytotoxicity. ECT has been shown to be effective in treating cutaneous and subcutaneous tumors, with response rates ranging from 70% to 90%. In some cases, ECT has been used to treat internal tumors, such as liver and pancreatic cancer, with promising results. While ECT is generally well-tolerated, potential side effects include pain, skin irritation, and muscle contractions. Long-term follow-up studies are needed to assess the durability of the response and the potential for late complications.
5.2. Gene Therapy
Electroporation-mediated gene therapy holds great promise for treating a variety of genetic diseases. Gene therapy involves the delivery of therapeutic genes into the patient’s cells to correct the underlying genetic defect. Electroporation can be used to deliver genes into various tissues, including muscle, skin, and liver. Clinical trials have demonstrated the safety and efficacy of electroporation-mediated gene therapy for treating diseases such as cystic fibrosis, muscular dystrophy, and hemophilia. However, several challenges remain, including the need to improve the efficiency of gene delivery and the duration of gene expression. Furthermore, there are ongoing concerns about the potential for insertional mutagenesis, which is the disruption of host cell genes by the delivered therapeutic gene. The risk of insertional mutagenesis can be minimized by using self-inactivating viral vectors or by targeting the delivery of the therapeutic gene to specific genomic locations using gene editing technologies such as CRISPR-Cas9.
5.3. Transdermal Drug Delivery
Electroporation has shown promise as a method for enhancing the transdermal delivery of drugs. Transdermal drug delivery offers several advantages over conventional drug administration routes, including improved patient compliance, reduced pain, and avoidance of first-pass metabolism. Electroporation can increase the permeability of the skin to a wide range of drugs, including small molecules, peptides, and proteins. Clinical studies have demonstrated the safety and efficacy of electroporation-enhanced transdermal drug delivery for treating conditions such as pain, diabetes, and skin disorders. However, the effectiveness of electroporation-enhanced transdermal drug delivery depends on several factors, including the properties of the drug, the skin hydration level, and the electric field parameters. Further research is needed to optimize electroporation protocols for specific drugs and skin conditions.
5.4 ReCET Therapy and Beyond
The ReCET therapy mentioned involves using electroporation to permeabilize cells in the duodenum. The intent is to regenerate the duodenal lining and improve glucose homeostasis. While initial results are promising, extensive clinical trials with long-term follow-up are necessary to establish the sustained efficacy and safety of this approach. The potential for long-term side effects, such as fibrosis or unintended damage to surrounding tissues, needs to be carefully evaluated. While ReCET targets a specific area in diabetes treatment, understanding its long-term effects is crucial for its viability. In addition, these investigations will pave the way for the expanded use of electroporation in other similar applications.
5.5 Immune Response
One of the less explored areas is the impact of electroporation on the immune system. Electroporation can trigger the release of intracellular molecules, such as ATP and DNA, which can act as danger-associated molecular patterns (DAMPs) and activate the innate immune system. This can lead to inflammation and potentially affect the therapeutic outcome. Depending on the specific context, the immune response can be either beneficial or detrimental. For example, in cancer therapy, the activation of the immune system can enhance the anti-tumor effect. In gene therapy, however, the immune response can lead to the rejection of the gene-modified cells. Therefore, it is important to understand the mechanisms by which electroporation activates the immune system and to develop strategies to modulate the immune response for optimal therapeutic benefit. Strategies to modulate the immune response could include the use of immunosuppressants or the incorporation of immune-modulatory molecules into the electroporation protocol.
Many thanks to our sponsor Esdebe who helped us prepare this research report.
6. Future Directions and Challenges
Electroporation has emerged as a versatile and powerful tool with broad applications in biotechnology and medicine. However, several challenges remain that need to be addressed to fully realize its potential. Some of the key future directions include:
- Improved Understanding of Mechanisms: Further research is needed to elucidate the complex mechanisms underlying electroporation, particularly the dynamics of pore formation and closure, the role of membrane proteins, and the cellular response to electric fields. This knowledge will enable the development of more efficient and selective electroporation protocols.
- Development of Novel Electrode Designs: The design of electrodes plays a critical role in determining the distribution of the electric field and the effectiveness of electroporation. Novel electrode designs, such as microelectrodes and nanoelectrodes, offer the potential for more precise and localized electroporation. These advanced electrode designs could potentially improve the efficacy of electroporation in hard-to-reach tissues.
- Optimization of Pulse Parameters: The optimal pulse parameters for electroporation depend on several factors, including the cell type, the target molecule, and the desired outcome. Machine learning algorithms could be used to optimize the pulse parameters for specific applications.
- Integration with Nanotechnology: The combination of electroporation with nanotechnology offers exciting opportunities for targeted drug and gene delivery. Nanoparticles can be used to encapsulate therapeutic molecules and deliver them specifically to target cells. Electroporation can then be used to enhance the uptake of the nanoparticles by the cells.
- Long-Term Safety Monitoring: Long-term follow-up studies are needed to assess the safety and efficacy of electroporation in various medical applications. These studies should focus on evaluating the potential for long-term side effects, such as fibrosis, inflammation, and immune responses. The lack of extensive long-term data remains a critical hurdle to overcome for widespread adoption.
- Standardization of Protocols: A lack of standardization in electroporation protocols makes it difficult to compare results across different studies and to translate research findings into clinical practice. Efforts should be made to develop standardized electroporation protocols for specific applications.
By addressing these challenges and pursuing these future directions, electroporation can be further developed into a safe, effective, and versatile tool for a wide range of medical applications.
Many thanks to our sponsor Esdebe who helped us prepare this research report.
7. Conclusion
Electroporation represents a significant advancement in the fields of biotechnology and medicine, offering a powerful method for manipulating cell membrane permeability for diverse applications. While substantial progress has been made in understanding the underlying mechanisms and optimizing the technology, several challenges remain. These include the need for a deeper understanding of long-term effects, improved targeting strategies, and standardization of protocols. Further research and development in these areas will pave the way for wider adoption of electroporation-based therapies and diagnostic tools, ultimately leading to improved patient outcomes.
Many thanks to our sponsor Esdebe who helped us prepare this research report.
References
- Neumann, E., Schaefer-Ridder, M., Wang, Y., & Hofschneider, P. H. (1982). Gene transfer into mouse lyoma cells by electroporation in high electric fields. The EMBO Journal, 1(7), 841–845.
- Weaver, J. C. (1993). Electroporation: a general introduction. Bioelectrochemistry and Bioenergetics, 30, 1–13.
- Gehl, J. (2003). Electroporation: applications in oncology. Acta Oncologica, 42(5-6), 437–451.
- Mir, L. M., Belehradek, J., Jr, Domenge, C., Orlowski, S., Poddevin, B., Belehradek, M., Scherman, D., & Paoletti, C. (1998). Electrochemotherapy, a novel antitumor treatment: first clinical transfer. Comptes Rendus de l’Académie des Sciences – Series III – Sciences de la Vie, 321(2-3), 101–107.
- Golzio, M., Teissié, J., & Rols, M. P. (2002). Direct visualization at the single-cell level of electrically mediated gene delivery. Proceedings of the National Academy of Sciences of the United States of America, 99(3), 1292–1297.
- Heller, R., Gilbert, R., & Martin, L. F. (2020). Electrochemotherapy: A Concise Review of the Evidence, Translational Oncology, 13(9), 100780
- Escoffre, J. M., Portet, T., Novès, M., Rols, M. P., & Golzio, M. (2009). Parameters for macromolecule electrotransfer into cells. Molecular Biotechnology, 41(2), 286–295.
- Pavšelj, N., Kandušer, M., Reberšek, M., Miklavčič, D., & Pucihar, G. (2019). Electroporation of cell membranes: the biophysical mechanisms of induced transport. Journal of Membrane Biology, 252(4), 427–441.
- Lusic, H., & Biasutto, L. (2020). Electroporation-Based Treatments in Oncology. Cancers, 12(11), 3487.
- Daud, A., Hajjari, M., Abu-Ghazaleh, R., & Mousa, S.A. (2021). Electroporation-based strategies for transdermal drug delivery. Journal of Controlled Release, 330, 254-267.
- Rosazza, C., Toffoli, G., Soden, D. M., & Rotoli, D. (2011). Gene electrotransfer: applications and clinical perspectives. Expert Opinion on Biological Therapy, 11(11), 1409–1424.
This report highlights the exciting potential of electroporation in ReCET therapy. I’m curious about research exploring the optimization of pulse parameters for different tissue types within the duodenum to maximize therapeutic effect while minimizing potential damage to adjacent organs.
Thanks for your comment! It’s a great point about optimizing pulse parameters for ReCET therapy in the duodenum. Research into tissue-specific parameters is crucial – considering factors like tissue thickness and conductivity could really refine the treatment and improve outcomes, offering greater precision and minimizing potential side effects.
Editor: MedTechNews.Uk
Thank you to our Sponsor Esdebe
The discussion of immune response post-electroporation is particularly compelling. Exploring the potential to harness this response for enhanced therapeutic outcomes, perhaps through controlled co-administration of immunomodulatory agents, could significantly broaden electroporation’s applications.
Thanks for highlighting the immune response! It’s definitely an area ripe for exploration. I wonder if anyone is investigating personalized electroporation protocols, tailoring pulse parameters based on an individual’s immune profile to optimize the therapeutic effect and minimize adverse reactions. It could be the next frontier in precision medicine with electroporation!
Editor: MedTechNews.Uk
Thank you to our Sponsor Esdebe
Electroporation manipulating cell membranes…sounds like cellular redecorating! Imagine offering cells a new “open-door” policy. Any chance we could use this tech to deliver tiny furniture (organelles) upgrades too? Now that would be a real cell makeover!
That’s a fantastic analogy! “Cellular redecorating” really captures the essence of it. The idea of delivering organelle upgrades is intriguing! While directly transplanting organelles is challenging, research explores delivering the *building blocks* and instructions for cells to rebuild and improve their own “furniture.” It’s like giving them an instruction manual and a DIY kit!
Editor: MedTechNews.Uk
Thank you to our Sponsor Esdebe