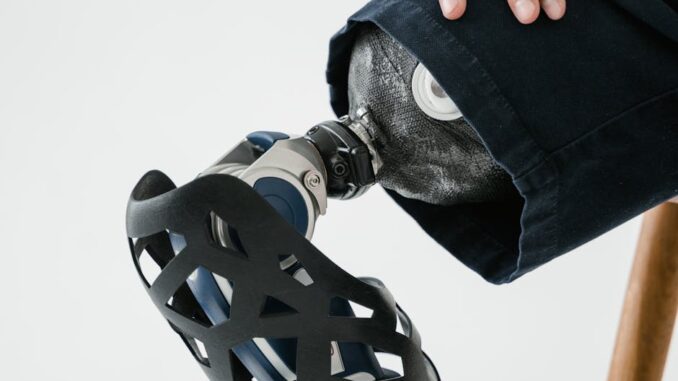
Abstract
Exoskeletons, wearable robotic devices designed to augment human capabilities or assist with rehabilitation, have witnessed significant advancements in recent years. This report provides a comprehensive review of exoskeleton technology, encompassing its historical development, diverse designs, control strategies, and applications across various domains, including medical rehabilitation, industrial augmentation, and military applications. Furthermore, the report delves into the challenges associated with exoskeleton adoption, such as power consumption, control complexity, and user acceptance. Finally, it explores future directions in exoskeleton research, focusing on emerging technologies like soft robotics, artificial intelligence integration, and personalized exoskeleton designs. This review aims to provide a holistic understanding of the current state-of-the-art in exoskeleton technology and highlight potential avenues for future innovation.
Many thanks to our sponsor Esdebe who helped us prepare this research report.
1. Introduction
The concept of exoskeletons, originating from science fiction, has transitioned into a tangible reality, driven by advancements in robotics, materials science, and control engineering. An exoskeleton can be defined as a wearable electromechanical device designed to enhance the physical capabilities of the user or assist with specific tasks. These devices operate by providing external support and power to the wearer’s limbs, effectively amplifying their strength, endurance, or precision. The development of exoskeletons has been fueled by diverse needs across various sectors. In the medical field, exoskeletons are employed for rehabilitation, assisting individuals with mobility impairments due to stroke, spinal cord injury, or other neurological conditions. In the industrial sector, exoskeletons are used to reduce physical strain and fatigue among workers performing repetitive or physically demanding tasks. Military applications include enhancing soldier performance by increasing load-carrying capacity and reducing the risk of injury.
The history of exoskeletons dates back to the 19th century with early attempts focusing on pneumatic and mechanical amplification. However, the practical realization of exoskeletons only became possible with the advent of powerful and compact actuators, advanced sensors, and sophisticated control algorithms. The evolution of exoskeleton technology can be broadly categorized into three generations: first-generation devices were primarily pneumatically or hydraulically powered and lacked sophisticated control; second-generation exoskeletons introduced electric actuators and basic control systems, allowing for more precise movement; and third-generation exoskeletons incorporate advanced sensors, sophisticated control algorithms (including artificial intelligence), and improved materials, enabling more natural and adaptable assistance. This review provides a detailed analysis of the current state of exoskeleton technology, examining its diverse applications, challenges, and future prospects.
Many thanks to our sponsor Esdebe who helped us prepare this research report.
2. Exoskeleton Designs and Classification
Exoskeletons can be categorized based on various factors, including the body part they augment (lower limb, upper limb, full-body), their power source (powered, passive), and their control strategy (pre-programmed, adaptive). A comprehensive understanding of these classifications is crucial for selecting the appropriate exoskeleton for a specific application.
2.1. Body Region Targeted
-
Lower Limb Exoskeletons: These are among the most prevalent types of exoskeletons, designed to assist with walking, standing, and sitting. They are widely used in rehabilitation for individuals with spinal cord injuries or stroke and in industrial settings to reduce fatigue associated with prolonged standing or heavy lifting. Lower limb exoskeletons can be further classified based on the joints they actuate (hip, knee, ankle) and the level of assistance they provide.
-
Upper Limb Exoskeletons: These are designed to augment or rehabilitate arm and hand function. They find applications in stroke rehabilitation, assisting individuals with grasping and manipulating objects, and in industrial settings for reducing strain during repetitive tasks. Upper limb exoskeletons often require more complex control strategies due to the higher degrees of freedom in the arm and hand.
-
Full-Body Exoskeletons: These exoskeletons augment the entire body, providing assistance for both the upper and lower limbs. They are typically used in military applications to increase load-carrying capacity and endurance. Full-body exoskeletons require sophisticated control systems to coordinate movement and maintain balance.
2.2. Power Source
-
Powered Exoskeletons: These exoskeletons utilize actuators (electric motors, pneumatic cylinders, or hydraulic systems) to provide assistance. They can generate significant force and are suitable for assisting individuals with significant motor impairments or for augmenting strength in industrial and military applications. However, powered exoskeletons require a power source (battery or external power supply), which can add weight and limit mobility.
-
Passive Exoskeletons: These exoskeletons do not use actuators to generate force. Instead, they rely on springs, dampers, and other mechanical elements to store and release energy, providing assistance by redirecting forces and reducing joint stress. Passive exoskeletons are lighter and require no power source, but they provide less assistance than powered exoskeletons. They are often used in industrial settings to reduce fatigue and prevent injuries.
2.3. Control Strategy
-
Pre-programmed Control: This type of control relies on pre-defined movement patterns. The exoskeleton follows a predetermined trajectory, regardless of the user’s intention. This approach is simple to implement but lacks adaptability to different users or tasks. It is often used in early-stage rehabilitation.
-
Adaptive Control: This type of control uses sensors to detect the user’s intention and adjusts the exoskeleton’s assistance accordingly. Adaptive control strategies can be further categorized as force-based control, position-based control, or impedance control. Force-based control uses force sensors to measure the user’s effort and provides assistance proportional to the applied force. Position-based control uses position sensors to track the user’s movement and provides assistance to maintain the desired trajectory. Impedance control combines force and position feedback to provide assistance that is both compliant and accurate. Adaptive control allows for more natural and intuitive movement, but it requires sophisticated sensors and control algorithms.
-
AI-Driven Control: Emerging control strategies leverage artificial intelligence (AI) and machine learning (ML) to personalize and optimize exoskeleton assistance. These systems can learn from the user’s movement patterns and adapt the control parameters in real-time to improve performance and comfort. AI-driven control holds significant promise for improving the effectiveness of exoskeletons in rehabilitation and other applications.
Many thanks to our sponsor Esdebe who helped us prepare this research report.
3. Applications of Exoskeletons
Exoskeletons have found diverse applications across various domains, demonstrating their versatility and potential to address a wide range of needs.
3.1. Medical Rehabilitation
Exoskeletons have emerged as a promising tool for rehabilitation following stroke, spinal cord injury, and other neurological conditions. By providing external support and assistance, exoskeletons can enable individuals with mobility impairments to perform movements that would otherwise be impossible. This can improve motor recovery, increase strength, and reduce spasticity. Studies have shown that exoskeleton-assisted training can lead to significant improvements in walking speed, balance, and functional independence in stroke survivors. In spinal cord injury, exoskeletons can enable individuals to stand and walk, improving cardiovascular health and reducing the risk of pressure sores. Furthermore, the use of virtual reality (VR) environments in conjunction with exoskeletons can enhance engagement and motivation during rehabilitation, leading to better outcomes. The integration of biofeedback and neurofeedback techniques can also personalize the rehabilitation process and optimize motor learning.
3.2. Industrial Augmentation
In the industrial sector, exoskeletons are used to reduce physical strain and fatigue among workers performing repetitive or physically demanding tasks. These devices can provide support for the back, shoulders, and legs, reducing the risk of musculoskeletal injuries. Exoskeletons can also increase productivity by allowing workers to perform tasks more efficiently and with less effort. Examples of industrial applications include assembly line work, construction, and logistics. Passive exoskeletons are particularly well-suited for industrial applications due to their lightweight and power-free operation. However, powered exoskeletons are increasingly being used for tasks that require significant strength augmentation.
3.3. Military Applications
Exoskeletons have significant potential to enhance soldier performance in military operations. These devices can increase load-carrying capacity, reduce fatigue, and improve endurance. Exoskeletons can also provide protection from injury and enhance mobility in challenging terrains. Military exoskeletons are typically full-body systems that provide assistance to both the upper and lower limbs. They require robust designs, high power density, and sophisticated control systems. The development of military exoskeletons is driven by the need to improve soldier survivability and effectiveness in combat.
3.4. Assistive Technology
Exoskeletons can serve as assistive devices for individuals with disabilities, enabling them to perform activities of daily living that would otherwise be impossible. For example, exoskeletons can assist individuals with paraplegia to stand and walk, improving their independence and quality of life. Exoskeletons can also assist individuals with upper limb impairments to perform tasks such as eating, dressing, and writing. The development of affordable and user-friendly exoskeletons is crucial for increasing accessibility to this technology for individuals with disabilities.
Many thanks to our sponsor Esdebe who helped us prepare this research report.
4. Control Strategies and Sensory Feedback
The effectiveness of an exoskeleton hinges on its control system and the sensory feedback it receives. Sophisticated control algorithms are required to ensure that the exoskeleton moves in a coordinated and natural manner, while sensory feedback is essential for adapting the assistance to the user’s needs and preventing injury.
4.1. Control Architectures
-
Centralized Control: In this architecture, a single controller manages all aspects of the exoskeleton’s movement. This approach simplifies coordination but can be computationally intensive and less robust to sensor failures.
-
Decentralized Control: In this architecture, multiple controllers manage individual joints or segments of the exoskeleton. This approach is more modular and robust to sensor failures, but it requires careful coordination between the different controllers.
-
Hybrid Control: This architecture combines the advantages of centralized and decentralized control. For example, a central controller might manage the overall gait pattern, while local controllers manage individual joint movements.
4.2. Sensory Feedback Mechanisms
-
Force/Torque Sensors: These sensors measure the forces and torques exerted by the user on the exoskeleton. This information can be used to estimate the user’s intention and adjust the assistance accordingly. Force/torque sensors are typically placed at the joints of the exoskeleton.
-
Position/Velocity Sensors: These sensors measure the position and velocity of the exoskeleton’s joints. This information can be used to track the user’s movement and ensure that the exoskeleton follows the desired trajectory. Position/velocity sensors can be encoders, potentiometers, or inertial measurement units (IMUs).
-
Electromyography (EMG) Sensors: These sensors measure the electrical activity of the user’s muscles. This information can be used to estimate the user’s intention and provide assistance that is synchronized with the user’s muscle activity. EMG sensors are typically placed on the skin over the muscles of interest.
-
Insole Pressure Sensors: These sensors measure the pressure distribution under the user’s feet. This information can be used to detect gait events, such as heel strike and toe-off, and adjust the exoskeleton’s assistance accordingly. Insole pressure sensors can also be used to detect balance problems and prevent falls.
4.3. Advanced Control Algorithms
-
Adaptive Impedance Control: This control strategy adjusts the impedance of the exoskeleton to match the user’s needs. Impedance is a measure of the exoskeleton’s resistance to movement. By adjusting the impedance, the exoskeleton can provide assistance that is both compliant and accurate. Adaptive impedance control requires sophisticated algorithms that can estimate the user’s intention and adjust the impedance parameters in real-time.
-
Reinforcement Learning: This machine learning technique can be used to train the exoskeleton to provide optimal assistance for a given task. Reinforcement learning algorithms learn from trial and error, gradually improving the exoskeleton’s performance over time. Reinforcement learning requires a significant amount of training data, but it can lead to significant improvements in performance.
-
Model Predictive Control (MPC): MPC uses a mathematical model of the exoskeleton and the user to predict the future behavior of the system. This information is then used to optimize the control inputs to achieve a desired goal, such as minimizing energy consumption or maximizing stability. MPC can handle complex dynamics and constraints, making it well-suited for controlling exoskeletons.
Many thanks to our sponsor Esdebe who helped us prepare this research report.
5. Challenges and Limitations
Despite the significant advancements in exoskeleton technology, several challenges and limitations remain that hinder their widespread adoption.
5.1. Power Consumption
Powered exoskeletons require a significant amount of power to operate, which limits their battery life and mobility. Improving the energy efficiency of exoskeletons is a major challenge. This can be achieved through the use of more efficient actuators, lighter materials, and optimized control algorithms. Energy harvesting techniques, such as regenerative braking, can also be used to recover energy during movement.
5.2. Control Complexity
Controlling an exoskeleton is a complex task, especially for individuals with motor impairments. Sophisticated control algorithms are required to ensure that the exoskeleton moves in a coordinated and natural manner. Developing intuitive and user-friendly control interfaces is crucial for increasing user acceptance. The use of AI and ML can help to personalize the control system and adapt it to the user’s individual needs.
5.3. Weight and Size
Exoskeletons can be bulky and heavy, which can limit their comfort and mobility. Reducing the weight and size of exoskeletons is a major challenge. This can be achieved through the use of lighter materials, such as carbon fiber and titanium, and more compact actuators. The development of soft exoskeletons, which use flexible materials and inflatable actuators, offers a promising approach to reducing weight and improving comfort.
5.4. Cost
Exoskeletons are currently expensive, which limits their accessibility to many individuals and organizations. Reducing the cost of exoskeletons is crucial for increasing their widespread adoption. This can be achieved through the use of more affordable materials, simpler designs, and mass production techniques. Open-source exoskeleton designs can also help to reduce costs by allowing researchers and developers to share their knowledge and expertise.
5.5. Safety
Ensuring the safety of exoskeleton users is paramount. Exoskeletons must be designed to prevent falls, collisions, and other injuries. Safety features such as emergency stop buttons, collision sensors, and gait monitoring systems are essential. User training is also crucial for ensuring safe and effective use of exoskeletons.
5.6. User Acceptance
User acceptance is a critical factor in the success of exoskeletons. Exoskeletons must be comfortable, easy to use, and aesthetically appealing. Addressing user concerns about weight, appearance, and social stigma is crucial for increasing user adoption. Involving users in the design process can help to ensure that exoskeletons meet their needs and preferences.
Many thanks to our sponsor Esdebe who helped us prepare this research report.
6. Future Directions
The future of exoskeleton technology is bright, with ongoing research and development focused on addressing the current challenges and limitations and exploring new applications. Key areas of future research include:
6.1. Soft Robotics
Soft robotics, which utilizes flexible materials and inflatable actuators, offers a promising approach to developing lighter, more comfortable, and more adaptable exoskeletons. Soft exoskeletons can conform to the user’s body and provide assistance without restricting movement. They are particularly well-suited for rehabilitation and assistive applications.
6.2. Artificial Intelligence Integration
The integration of AI and ML can significantly improve the performance and adaptability of exoskeletons. AI can be used to personalize the control system, optimize assistance, and predict the user’s intention. AI can also be used to analyze sensor data and detect potential problems, such as falls or collisions. AI-driven exoskeletons have the potential to revolutionize rehabilitation, industrial augmentation, and other applications.
6.3. Personalized Exoskeleton Designs
Tailoring exoskeletons to the individual user’s needs and preferences is crucial for maximizing their effectiveness and comfort. Personalized exoskeleton designs can be achieved through the use of 3D printing, adjustable components, and adaptive control algorithms. This approach allows for the creation of exoskeletons that fit the user perfectly and provide the optimal level of assistance.
6.4. Integration with Virtual Reality Environments
Integrating exoskeletons with VR environments can enhance engagement and motivation during rehabilitation. VR can provide realistic simulations of real-world tasks, allowing users to practice their skills in a safe and controlled environment. VR can also provide feedback on performance and motivate users to improve their skills.
6.5. Advanced Materials
The development of advanced materials, such as lightweight composites, shape-memory alloys, and self-healing polymers, can significantly improve the performance and durability of exoskeletons. These materials can reduce weight, increase strength, and improve resistance to wear and tear.
Many thanks to our sponsor Esdebe who helped us prepare this research report.
7. Ethical Considerations
The development and use of exoskeletons raise several ethical considerations that must be addressed. These include:
7.1. Job Displacement
The use of exoskeletons in industrial settings could lead to job displacement as workers become more productive and fewer workers are needed. This raises concerns about the social and economic impact of exoskeleton technology.
7.2. Privacy
Exoskeletons collect data about the user’s movement and activity, which could be used to track their location, monitor their health, or assess their performance. This raises concerns about the privacy of exoskeleton users.
7.3. Augmentation Inequality
The benefits of exoskeleton technology may not be equally distributed, potentially exacerbating existing inequalities. Affluent individuals or organizations may have access to more advanced and effective exoskeletons, while less affluent individuals or organizations may be left behind.
7.4. Psychological Impact
The use of exoskeletons could have psychological impacts on users, such as feelings of dependence, reduced self-esteem, or altered body image. These issues need careful consideration in the design and implementation of exoskeleton technology. It is important to involve mental health professionals in the development process to address these concerns proactively.
Many thanks to our sponsor Esdebe who helped us prepare this research report.
8. Conclusion
Exoskeleton technology has made significant strides in recent years, demonstrating its potential to enhance human capabilities and assist with rehabilitation. However, several challenges remain that must be addressed before exoskeletons can achieve widespread adoption. These include reducing power consumption, improving control complexity, reducing weight and size, lowering cost, ensuring safety, and increasing user acceptance. Future research should focus on soft robotics, AI integration, personalized designs, VR integration, and advanced materials. Furthermore, it is crucial to address the ethical considerations associated with exoskeleton technology, such as job displacement, privacy, augmentation inequality, and psychological impact. By addressing these challenges and exploring new avenues for innovation, exoskeleton technology can revolutionize various fields, improving the lives of individuals with disabilities, enhancing worker productivity, and augmenting soldier performance.
Many thanks to our sponsor Esdebe who helped us prepare this research report.
References
- Dollar, A. M., & Herr, H. (2008). Lower extremity exoskeletons and active orthoses: Challenges and state-of-the-art. IEEE Transactions on Robotics, 24(1), 144-158.
- Esquenazi, A., & Packel, A. (2012). Clinical application of robotics in rehabilitation. American Journal of Physical Medicine & Rehabilitation, 91(11 Suppl 3), S153-S167.
- Kazerooni, H. (2012). Human power augmentation by robots. International Journal of Humanoid Robotics, 9(01), 1-19.
- Kolakowsky-Hayner, S. A., et al. (2023). Upper limb exoskeletons for rehabilitation after stroke: A systematic review. Journal of NeuroEngineering and Rehabilitation, 20(1), 1-23.
- Pons, J. L. (Ed.). (2008). Wearable robots: biomechatronic exoskeletons. John Wiley & Sons.
- Riener, R., Lünenburger, L., & Colombo, G. (2006). Human–machine interface for robot-aided rehabilitation. Rehabilitation Robotics, 17-47.
- Varghese, R. J., et al. (2015). Lower extremity exoskeletons for gait training in neurological conditions: A systematic review and meta-analysis. Topics in Stroke Rehabilitation, 22(6), 569-582.
- Young, A. J., Ferris, D. P., & Remy, C. D. (2015). Bridging the gap between laboratory and community: Wearable robotic exoskeletons for daily life assistance. Journal of NeuroEngineering and Rehabilitation, 12(1), 1-13.
- Zoss, A., Kazerooni, H., & Chu, A. (2006). Biomechanical model identification of human walking for the design of exoskeletons. IEEE Transactions on Robotics, 22(5), 980-991.
This report effectively highlights the diverse applications of exoskeletons, especially in rehabilitation. The potential of AI-driven control to personalize and optimize assistance seems particularly promising for enhancing user outcomes and warrants further exploration.
Thanks for your insightful comment! The AI integration aspect is truly exciting. We’re seeing potential for exoskeletons to adapt in real-time to a user’s unique needs, making rehabilitation far more effective. Further research in areas such as predictive algorithms could unlock even greater benefits. What are your thoughts on the ethical implications of AI-driven exoskeletons?
Editor: MedTechNews.Uk
Thank you to our Sponsor Esdebe