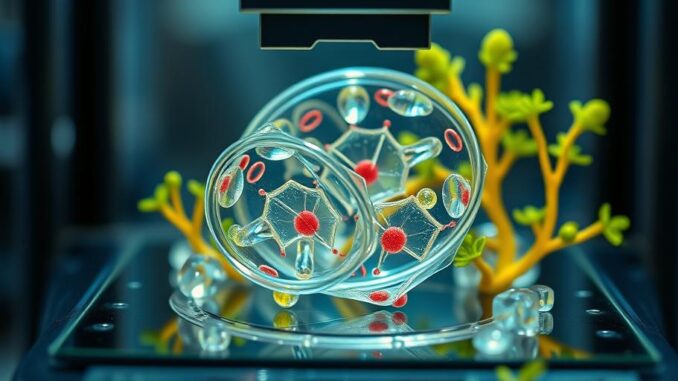
Abstract
Bioprinting, a rapidly evolving field at the intersection of additive manufacturing and tissue engineering, holds immense promise for creating functional tissues and organs. While various bioprinting techniques exist, FRESH (Freeform Reversible Embedding of Suspended Hydrogels) bioprinting has emerged as a particularly powerful approach. This report provides a comprehensive overview of FRESH bioprinting, focusing on its underlying principles, advantages, limitations, and recent advancements. It explores the critical role of support baths in FRESH, the design considerations for bioinks, and the challenges associated with achieving long-term cell viability and tissue functionality. Beyond a review of the core technology, this report delves into the synergy between FRESH bioprinting and advanced materials science, highlighting how the integration of novel biomaterials, including nanocomposites and stimuli-responsive polymers, is expanding the scope of FRESH and enabling the fabrication of increasingly complex and sophisticated tissue constructs. The potential of FRESH bioprinting to revolutionize regenerative medicine, drug discovery, and personalized medicine is discussed, alongside considerations for future research directions and clinical translation.
Many thanks to our sponsor Esdebe who helped us prepare this research report.
1. Introduction
Regenerative medicine aims to restore or replace damaged tissues and organs, offering potential solutions for a wide range of debilitating conditions. Bioprinting, an additive manufacturing technique that uses bioinks containing cells, biomaterials, and growth factors, represents a significant advancement in this field. Unlike traditional tissue engineering approaches that rely on static scaffolds, bioprinting allows for precise control over cell placement and scaffold architecture, enabling the creation of three-dimensional (3D) tissue constructs with tailored properties [1].
Several bioprinting techniques have been developed, each with its own advantages and limitations. Extrusion-based bioprinting, inkjet bioprinting, and laser-assisted bioprinting are among the most common methods [2]. However, many of these techniques struggle to maintain the structural integrity of complex constructs, particularly those composed of soft hydrogels that lack inherent mechanical strength. This limitation has spurred the development of support-based bioprinting methods, with FRESH bioprinting standing out as a leading technique.
FRESH bioprinting, pioneered by Feinberg and his team at Carnegie Mellon University, addresses the structural challenges by embedding the bioprinted construct within a support bath [3]. This support bath, typically composed of a yield-stress material such as gelatin microparticles, provides temporary mechanical support during the printing process, allowing for the fabrication of complex and delicate structures that would otherwise collapse under their own weight. The support bath can then be easily removed by heating, leaving behind the bioprinted tissue construct. This method has shown promise in creating complex tissue structures, however, the support bath itself can sometimes be difficult to remove completely without damaging the printed part.
This report aims to provide a comprehensive overview of FRESH bioprinting, focusing not only on the core technology but also on its intersection with advanced materials science. The integration of novel biomaterials, including nanocomposites and stimuli-responsive polymers, is revolutionizing the capabilities of FRESH, enabling the creation of more functional and physiologically relevant tissue constructs. The report will discuss the challenges and opportunities associated with this integration, as well as the potential of FRESH bioprinting to transform regenerative medicine and related fields.
Many thanks to our sponsor Esdebe who helped us prepare this research report.
2. Principles of FRESH Bioprinting
The core principle of FRESH bioprinting is to provide a temporary support environment that allows for the precise deposition of bioinks without compromising structural integrity. This is achieved through the use of a yield-stress support bath, which exhibits solid-like behavior at rest but flows under applied stress, allowing the bioprinting nozzle to move freely. The support bath also prevents the bioink from spreading or collapsing before it can be crosslinked, thereby ensuring accurate replication of the desired structure [4].
2.1 Support Bath Materials
The most common support bath material used in FRESH bioprinting is gelatin microparticles. Gelatin is a denatured form of collagen, a naturally occurring protein found in the extracellular matrix (ECM). Gelatin microparticles are biocompatible, biodegradable, and relatively inexpensive, making them an attractive option for bioprinting applications. Importantly, they can be easily removed by raising the temperature above gelatin’s melting point, allowing the bioprinted construct to be released from the support bath without significant damage [5].
However, other support bath materials have also been explored. These include Pluronic F-127, a thermoresponsive polymer that forms a gel at low temperatures and a liquid at room temperature [6]; Carbopol, a crosslinked polyacrylic acid polymer that exhibits shear-thinning behavior [7]; and even clay-based slurries. Each material offers unique advantages and disadvantages in terms of mechanical properties, biocompatibility, and ease of removal. For example, while Pluronic F-127 can be removed easily, it provides less mechanical support compared to gelatin microparticles.
The choice of support bath material depends on the specific requirements of the bioprinting application. Factors to consider include the viscosity and crosslinking behavior of the bioink, the desired resolution and complexity of the construct, and the sensitivity of the cells to temperature and mechanical stress.
2.2 Bioink Design
The bioink is the key component of any bioprinting process, containing the cells, biomaterials, and growth factors necessary for tissue regeneration. In FRESH bioprinting, the bioink must be carefully designed to ensure compatibility with the support bath and to achieve the desired mechanical and biological properties [8].
Hydrogels are the most commonly used biomaterials in FRESH bioinks. Hydrogels are water-swollen polymers that mimic the ECM, providing a supportive environment for cells. Natural hydrogels, such as alginate, collagen, and fibrin, are biocompatible and biodegradable, but often lack the mechanical strength needed for load-bearing applications. Synthetic hydrogels, such as polyethylene glycol (PEG) and poly(vinyl alcohol) (PVA), offer greater control over mechanical properties and degradation rates, but may lack the cell-binding motifs found in natural hydrogels [9].
To overcome these limitations, researchers often combine natural and synthetic hydrogels to create hybrid bioinks with improved mechanical and biological properties. For example, combining alginate with cell-adhesive peptides can enhance cell attachment and proliferation. Similarly, incorporating growth factors into the bioink can promote cell differentiation and tissue maturation [10].
The viscosity and crosslinking behavior of the bioink are also critical considerations. The bioink must be viscous enough to maintain its shape during printing but must also be able to flow through the bioprinting nozzle without clogging. Crosslinking can be achieved through various mechanisms, including chemical crosslinking (e.g., using calcium chloride for alginate), enzymatic crosslinking (e.g., using transglutaminase for fibrin), and photo-crosslinking (e.g., using UV light for methacrylated hydrogels) [11].
2.3 FRESH Bioprinting Process
The FRESH bioprinting process typically involves the following steps:
- Preparation of the support bath: The support bath material (e.g., gelatin microparticles) is prepared and packed into a container.
- Preparation of the bioink: The bioink, containing cells, biomaterials, and growth factors, is prepared according to the desired formulation.
- Bioprinting: The bioprinting nozzle is moved through the support bath, depositing the bioink layer by layer according to the desired 3D model. The support bath provides temporary mechanical support, preventing the bioink from collapsing.
- Crosslinking: The bioink is crosslinked to solidify the structure. This can be achieved through various mechanisms, as discussed above.
- Support bath removal: The support bath is removed by raising the temperature (for gelatin microparticles) or by dissolving it in a suitable solvent (for other materials).
- Culture and maturation: The bioprinted construct is cultured in a bioreactor to promote cell proliferation, differentiation, and tissue maturation.
Many thanks to our sponsor Esdebe who helped us prepare this research report.
3. Advantages and Limitations of FRESH Bioprinting
3.1 Advantages
FRESH bioprinting offers several advantages over other bioprinting techniques:
- Enhanced Structural Fidelity: The support bath allows for the fabrication of complex and delicate structures that would otherwise be impossible to create. This is particularly important for tissues with intricate geometries, such as valves and branched vascular networks.
- Versatility in Bioink Selection: FRESH is compatible with a wide range of bioinks, including soft hydrogels, cell suspensions, and even cell aggregates. This versatility allows researchers to tailor the bioink to the specific requirements of the tissue being printed.
- High Cell Viability: The support bath provides a protective environment for cells during the printing process, minimizing shear stress and preventing cell damage. Studies have shown that FRESH bioprinting can achieve high cell viability, even with sensitive cell types [12].
- Scalability: FRESH bioprinting can be scaled up to produce larger tissue constructs, making it suitable for clinical applications.
3.2 Limitations
Despite its advantages, FRESH bioprinting also has some limitations:
- Support Bath Removal: Removing the support bath without damaging the bioprinted construct can be challenging, especially for delicate structures. Incomplete removal of the support material can also affect cell viability and tissue function. While optimisation of the removal process can overcome many of these issues it still requires consideration.
- Resolution: The resolution of FRESH bioprinting is limited by the size of the bioprinting nozzle and the particle size of the support bath material. Achieving high resolution requires the use of small nozzles and fine support particles, which can be technically challenging.
- Mechanical Properties: While the support bath provides temporary mechanical support, the mechanical properties of the bioprinted construct are ultimately determined by the bioink itself. Achieving the desired mechanical properties often requires the use of crosslinking agents or reinforcement materials, which can affect cell viability and biocompatibility.
- Vascularization: Creating functional vascular networks within bioprinted tissues remains a major challenge. Without adequate vascularization, cells in the interior of the construct can suffer from hypoxia and nutrient deprivation, leading to cell death and tissue necrosis. This remains one of the most significant challenges for all bioprinting technologies and FRESH is no exception. Whilst FRESH can be used to create vascular networks, ensuring they remain patent and functional is an ongoing area of research.
Many thanks to our sponsor Esdebe who helped us prepare this research report.
4. FRESH Bioprinting and Advanced Materials Science
The capabilities of FRESH bioprinting are being significantly enhanced through the integration of advanced materials science. The development of novel biomaterials with tailored properties is expanding the scope of FRESH and enabling the fabrication of increasingly complex and sophisticated tissue constructs.
4.1 Nanocomposites
Nanocomposites, which consist of a polymer matrix reinforced with nanoscale particles, offer a promising approach to improve the mechanical properties and biological functionality of bioinks. The incorporation of nanoparticles, such as carbon nanotubes, graphene oxide, and hydroxyapatite, can significantly enhance the strength, stiffness, and toughness of hydrogels [13].
For example, incorporating carbon nanotubes into a collagen-based bioink can increase its tensile strength and electrical conductivity, making it suitable for bioprinting cardiac tissues [14]. Similarly, incorporating hydroxyapatite nanoparticles into an alginate-based bioink can improve its osteoconductivity, making it suitable for bioprinting bone tissues [15].
In addition to improving mechanical properties, nanoparticles can also be used to deliver growth factors, drugs, and other bioactive molecules to cells. For example, nanoparticles can be loaded with growth factors and then incorporated into the bioink. As the nanoparticles degrade, they release the growth factors, promoting cell differentiation and tissue maturation [16].
4.2 Stimuli-Responsive Polymers
Stimuli-responsive polymers, also known as smart polymers, are materials that change their properties in response to external stimuli, such as temperature, pH, light, or magnetic fields. These materials can be used to create dynamic bioinks that can adapt to the changing needs of the tissue [17].
For example, thermoresponsive polymers, such as Pluronic F-127, can be used to create bioinks that solidify at body temperature and liquefy at lower temperatures. This property can be used to control the release of cells or drugs from the bioprinted construct. Similarly, pH-responsive polymers can be used to create bioinks that degrade in response to changes in pH, allowing for controlled degradation of the scaffold and release of growth factors [18].
Light-responsive polymers can be used to create bioinks that can be patterned with light, allowing for precise control over cell placement and scaffold architecture. For example, photo-crosslinkable hydrogels can be used to create complex 3D structures by selectively crosslinking the bioink with light [19].
4.3 4D Bioprinting
4D bioprinting takes the concept of stimuli-responsive polymers one step further, creating tissue constructs that can change their shape or function over time in response to external stimuli. This is achieved by incorporating stimuli-responsive materials into the bioink and then programming the construct to undergo a specific transformation upon exposure to the appropriate stimulus [20].
For example, a 4D bioprinted vascular graft could be designed to expand in response to increasing blood pressure, adapting to the changing needs of the patient. Similarly, a 4D bioprinted bone scaffold could be designed to degrade over time, allowing for the gradual replacement of the scaffold with natural bone tissue [21].
4D bioprinting is a relatively new field, but it holds immense potential for creating dynamic and functional tissue constructs that can adapt to the complex and changing environment of the human body.
Many thanks to our sponsor Esdebe who helped us prepare this research report.
5. Applications of FRESH Bioprinting
FRESH bioprinting has a wide range of potential applications in regenerative medicine, drug discovery, and personalized medicine.
5.1 Regenerative Medicine
In regenerative medicine, FRESH bioprinting can be used to create functional tissues and organs for transplantation. Several tissues and organs have already been bioprinted using FRESH, including:
- Cardiac Tissue: FRESH has been used to bioprint heart valves, myocardial patches, and even entire hearts. These bioprinted cardiac tissues can be used to repair damaged heart tissue or to replace diseased heart valves [22].
- Bone Tissue: FRESH has been used to bioprint bone scaffolds with complex geometries that can promote bone regeneration. These scaffolds can be used to repair bone defects or to replace damaged bone tissue [23].
- Cartilage Tissue: FRESH has been used to bioprint cartilage scaffolds that can promote cartilage regeneration. These scaffolds can be used to repair damaged cartilage in joints [24].
- Skin Tissue: FRESH has been used to bioprint skin grafts that can be used to treat burns and other skin injuries [25].
5.2 Drug Discovery
FRESH bioprinting can also be used to create 3D tissue models for drug screening. These 3D tissue models more accurately mimic the complexity of human tissues compared to traditional 2D cell cultures, providing a more physiologically relevant platform for drug testing [26].
For example, FRESH bioprinting can be used to create 3D tumor models that can be used to screen for new cancer drugs. These 3D tumor models can be used to study the effects of drugs on tumor growth, metastasis, and drug resistance [27].
5.3 Personalized Medicine
FRESH bioprinting can be used to create personalized tissues and organs for individual patients. This is achieved by using the patient’s own cells to create the bioink, eliminating the risk of immune rejection [28].
For example, FRESH bioprinting can be used to create personalized skin grafts for burn patients. These skin grafts can be used to cover large areas of burned skin and can significantly reduce the risk of infection and scarring [29].
Many thanks to our sponsor Esdebe who helped us prepare this research report.
6. Challenges and Future Directions
Despite the significant progress made in FRESH bioprinting, several challenges remain before it can be widely adopted for clinical applications:
- Vascularization: As mentioned earlier, creating functional vascular networks within bioprinted tissues remains a major challenge. Future research needs to focus on developing new strategies for vascularizing bioprinted tissues, such as incorporating microfluidic channels into the scaffold or using growth factors to stimulate angiogenesis. The recent integration of sacrificial printing techniques alongside FRESH offers promise in creating perfusable vascular networks, however, long term patency remains a challenge.
- Long-Term Cell Viability: Ensuring long-term cell viability within bioprinted tissues is also critical. Future research needs to focus on optimizing the bioink formulation, the culture conditions, and the scaffold architecture to promote cell survival and tissue maturation. Encapsulating cells in protective microenvironments or using bioreactors to provide nutrients and oxygen can also improve cell viability. The effects of long term culture on FRESH printed parts also needs consideration.
- Mechanical Properties: Achieving the desired mechanical properties for bioprinted tissues remains a challenge, particularly for load-bearing applications. Future research needs to focus on developing new biomaterials and crosslinking strategies that can improve the mechanical strength and toughness of bioprinted tissues. The use of nanocomposites and stimuli-responsive polymers offers a promising avenue for improving mechanical properties.
- Bioprinting Automation and Scalability: Improving the automation and scalability of FRESH bioprinting is essential for clinical translation. Future research needs to focus on developing automated bioprinting systems that can produce large numbers of tissue constructs with high reproducibility. The development of standardized bioprinting protocols and quality control measures is also crucial.
- Regulatory Approval: Obtaining regulatory approval for bioprinted tissues and organs is a major hurdle. Future research needs to focus on demonstrating the safety and efficacy of bioprinted products in preclinical and clinical trials. The development of clear regulatory guidelines for bioprinting is also essential.
In conclusion, FRESH bioprinting is a promising technology that holds immense potential for revolutionizing regenerative medicine, drug discovery, and personalized medicine. By addressing the remaining challenges and continuing to innovate in the areas of biomaterials, bioprinting techniques, and tissue engineering, FRESH bioprinting can pave the way for the creation of functional tissues and organs that can improve the lives of millions of people.
Many thanks to our sponsor Esdebe who helped us prepare this research report.
7. Conclusion
FRESH bioprinting stands as a significant advancement in the field of tissue engineering, offering a robust platform for creating complex and functional tissue constructs. Its unique ability to support the deposition of delicate bioinks, coupled with the growing integration of advanced materials science, is expanding the possibilities for regenerative medicine. While challenges remain in areas such as vascularization, long-term cell viability, and scalability, the ongoing research and development efforts are steadily pushing the boundaries of what is achievable. As the field matures and the regulatory landscape evolves, FRESH bioprinting is poised to play a transformative role in creating personalized therapies, accelerating drug discovery, and ultimately, improving human health.
Many thanks to our sponsor Esdebe who helped us prepare this research report.
References
[1] Murphy, S. V., & Atala, A. (2014). 3D bioprinting of tissues and organs. Nature biotechnology, 32(8), 773-785.
[2] Ozbolat, I. T. (2015). Scaffolding and bioprinting. Bioprinting, 1(1-2), 1-3.
[3] Lee, A., Hudson, A. R., Shiwarski, D. J., Tashman, J. W., Hinton, T. J., Yerneni, S., … & Feinberg, A. W. (2019). 3D bioprinting of collagen to rebuild components of the human heart. Science, 365(6452), 482-487.
[4] Hinton, T. J., Jaller, D., Park, J. H., Taniguchi, R., Polleux, J., Moon, T., & Feinberg, A. W. (2015). Three-dimensional printing of complex biological structures by freeform reversible embedding of suspended hydrogels. Science advances, 1(9), e1500758.
[5] Skylar-Scott, M. A., Mueller, J., Vellinger, L. A., Nguyen, P. K., Gleadall, A., & Lewis, J. A. (2019). Biomanufacturing of organ-specific tissues with complex microarchitecture. Science advances, 5(8), eaaw2459.
[6] Kolesky, D. B., Truby, R. L., Gladman, A. S., Busbee, T. A., Homan, K. A., & Lewis, J. A. (2014). 3D bioprinting of vascularized, heterogeneous cell-laden tissue constructs. Advanced Materials, 26(19), 3124-3130.
[7] Highley, C. B., Record, K. E., Burdick, J. A. (2015). Shear-thinning hydrogels for bioprinting. ACS Biomaterials Science & Engineering, 1(1), 4-15.
[8] Groll, J., Boland, T., Blunk, T., Burdick, J. A., Cho, D. W., Dalton, P. D., … & Zenobi-Wong, M. (2016). Bioinks for next-generation bioprinting: a review. Advanced Materials, 28(18), 3447-3468.
[9] Caló, E., & Khutoryanskiy, V. V. (2015). Biomedical applications of hydrogels: a review of patents and commercial products. European Polymer Journal, 65, 252-275.
[10] Matai, I., Kaur, G., Agarwal, S., & Kapoor, S. (2020). Progress in 3D bioprinting technology for tissue engineering and regenerative medicine. Biomaterials, 226, 119536.
[11] DeForest, S. H., Anseth, K. S. (2011). Cytocompatible click-based hydrogels with tunable degradation for adipose tissue engineering. Acta Biomaterialia, 7(5), 2172-2179.
[12] Tashman, J. W., Bonito, V., Shiwarski, D. J., Bhatia, S., & Feinberg, A. W. (2021). FRESH 2.0: Improved 3D bioprinting of collagen and other next-generation biomaterials. Matter, 4(1), 361-377.
[13] Bartnikowski, M., Ferreira, N. M., Rodrigues, G., Godinho, L. S., Sousa, R. A., Bernardo, A., … & Pashkuleva, I. (2018). Advanced bioinks for 3D bioprinting. Advanced healthcare materials, 7(11), 1701554.
[14] Shin, S. R., Aghaloo, T., Suzuki, R., Warner, S. M., Lyons, K. M., Urata, M. M., … & Khademhosseini, A. (2013). Nanoengineered cardiac patches for advanced cardiac regeneration. ACS nano, 7(2), 1680-1691.
[15] Chia, H. N., & Wu, B. M. (2015). The role of scaffold architecture and material composition in bone regeneration: A review. Biomaterials, 36, 229-248.
[16] Ho, P. P., Tay, C. Y., & Tan, L. P. (2015). Nanomaterials for growth factor delivery in tissue engineering. Journal of Materials Chemistry B, 3(4), 563-577.
[17] Stuart, M. A. C., Huck, W. T. S., Genzer, J., Müller, M., Ober, C., Stamm, M., … & Van Der Gucht, J. (2010). Responsive polymer materials: From fundamentals to applications. Nature materials, 9(2), 101-113.
[18] Hamidi, M., Azadi, A., & Rafiei, P. (2008). Hydrogel nanoparticles in drug delivery. Advanced drug delivery reviews, 60(15), 1638-1649.
[19] Fairman, R., Grinstaff, M. W., & Vunjak-Novakovic, G. (2018). Photopatterning biomaterials for tissue engineering. Advanced materials, 30(24), 1707783.
[20] Tibbits, S. (2014). 4D printing: Multi-material shape change. Architectural Design, 84(1), 116-121.
[21] Gladman, A. S., Matsumoto, E. A., Nuzzo, R. G., Mahadevan, L., & Lewis, J. A. (2016). Biomimetic 4D printing. Nature materials, 15(4), 413-418.
[22] Feinberg, A. W. (2021). Bioprinting a human heart. Trends in Biotechnology, 39(6), 543-545.
[23] Bose, S., Roy, M., & Bandyopadhyay, A. (2012). Recent advances in bone tissue engineering scaffolds. Trends in Biotechnology, 30(10), 546-554.
[24] Visser, J., Melchels, F. P., Jeon, J. E., Van Osch, G. J., & Dhert, W. J. (2013). Bioink properties and printing parameters for cartilage tissue engineering. Biofabrication, 5(2), 025005.
[25] Cubo, N., Garcia, M., del Cañizo, J. F., Velasco, D., & Jorcano, J. L. (2016). 3D bioprinting of functional human skin: production and in vivo analysis. Biofabrication, 9(1), 015006.
[26] Ingber, D. E. (2008). Mechanochemical switching between growth and differentiation during morphogenesis. Developmental cell, 14(4), 461-478.
[27] Ravi, M., Paramesh, V., Singamaneni, S., Kundu, B., Agrawal, A., & Bellamkonda, R. V. (2015). 3D cell culture systems: advantages and applications. Journal of cellular physiology, 230(1), 16-26.
[28] Atala, A. (2011). Tissue engineering. The Lancet, 378(9789), 474-476.
[29] Lee, J. W., Choi, Y. J., Lee, H., Park, J. K., Seo, Y. B., & Kim, B. S. (2017). 3D bioprinting of human skin with in vivo therapeutic potential. Scientific reports, 7(1), 1-11.
The report mentions challenges in vascularization of 3D printed tissues. What strategies, beyond microfluidic channels and growth factors, are being explored to promote angiogenesis and ensure long-term patency in these complex constructs, particularly regarding cell sourcing for vascular structures?
That’s a great question! Beyond microfluidics and growth factors, researchers are exploring co-printing with sacrificial materials to create perfusable networks. Cell sourcing is also key; some are investigating endothelial progenitor cells and induced pluripotent stem cells for more robust vascularization. The long-term stability is a big focus!
Editor: MedTechNews.Uk
Thank you to our Sponsor Esdebe
The report highlights the need for improved mechanical properties in bioprinted tissues. Have researchers explored the use of crosslinking strategies beyond traditional chemical methods, such as enzymatic or photo-crosslinking, to enhance the mechanical integrity and biocompatibility of FRESH-printed constructs?
That’s an excellent point! Indeed, enzymatic and photo-crosslinking are gaining traction. These methods often offer improved biocompatibility and finer control over the crosslinking process, which can lead to enhanced mechanical properties without compromising cell viability. Exploring these advanced crosslinking strategies is a key focus in the ongoing efforts to refine FRESH bioprinting. Thanks for raising this important aspect!
Editor: MedTechNews.Uk
Thank you to our Sponsor Esdebe