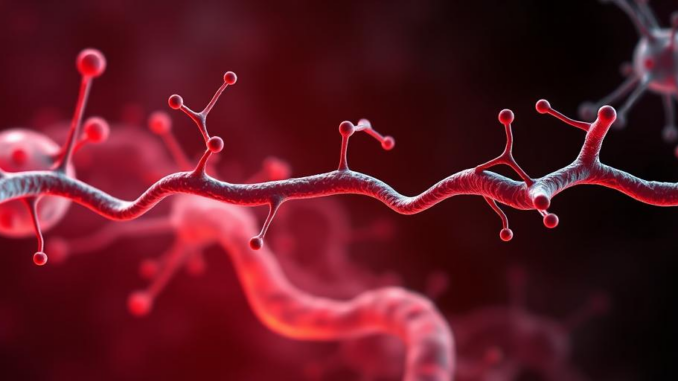
Abstract
Glycolysis, the primary pathway for glucose metabolism, is fundamentally altered in cancer cells compared to their normal counterparts. This shift, characterized by increased glucose uptake, enhanced lactate production even in the presence of oxygen (the Warburg effect), and altered expression of glycolytic enzymes, is a hallmark of malignant transformation. This report provides an in-depth exploration of the intricacies of glycolysis in cancer, extending beyond the well-established Warburg effect to analyze the nuanced roles of specific glycolytic enzymes and their impact on cancer hallmarks such as proliferation, metastasis, and therapy resistance. It further investigates the complex interplay between glycolysis and the tumor microenvironment, including the effects of increased lactate production on angiogenesis, immune evasion, and genomic instability. Finally, it critically evaluates the therapeutic potential of targeting glycolytic pathways, considering both the challenges and opportunities associated with this approach.
Many thanks to our sponsor Esdebe who helped us prepare this research report.
1. Introduction
Cancer cells exhibit distinct metabolic adaptations that support their uncontrolled proliferation, survival, and metastasis. While normal cells primarily rely on mitochondrial oxidative phosphorylation (OXPHOS) for energy production in the presence of oxygen, many cancer cells preferentially utilize glycolysis, even under normoxic conditions. This phenomenon, known as the Warburg effect or aerobic glycolysis, was first observed by Otto Warburg in the 1920s and has since been recognized as a fundamental characteristic of cancer metabolism [1]. Glycolysis, the breakdown of glucose into pyruvate, generates ATP and crucial metabolic intermediates that fuel anabolic pathways essential for cell growth and division. The subsequent conversion of pyruvate to lactate, even in the presence of oxygen, results in acidification of the tumor microenvironment. While initially perceived as an inefficient pathway, accumulating evidence suggests that the Warburg effect confers several advantages to cancer cells, including enhanced biomass production, redox balance maintenance, and immune evasion [2]. This report will delve into the complexities of glycolysis in cancer, exploring the roles of specific enzymes, the impact on the tumor microenvironment, and the therapeutic potential of targeting this pathway.
Many thanks to our sponsor Esdebe who helped us prepare this research report.
2. The Warburg Effect: Beyond Aerobic Glycolysis
The Warburg effect is not merely an increase in glycolytic rate; it encompasses a complex reprogramming of cellular metabolism. While enhanced glucose uptake and lactate production are key features, the Warburg effect also involves alterations in the expression and activity of glycolytic enzymes, as well as changes in mitochondrial function. Some cancer cells exhibit impaired mitochondrial OXPHOS, making them more reliant on glycolysis for ATP production. However, it’s crucial to recognize that not all cancer cells have defective mitochondria. In fact, many cancer cells maintain functional mitochondria and utilize both glycolysis and OXPHOS to meet their energy and biosynthetic demands, albeit with an altered balance [3].
Furthermore, the Warburg effect is not a uniform phenomenon across all cancer types. Different cancer subtypes exhibit varying degrees of glycolytic activity and reliance on OXPHOS. For instance, some highly aggressive tumors, such as certain subtypes of breast cancer and glioblastoma, exhibit a pronounced Warburg effect, while others may rely more heavily on OXPHOS or even exhibit metabolic plasticity, shifting between glycolysis and OXPHOS depending on nutrient availability and environmental conditions [4]. This heterogeneity underscores the importance of understanding the specific metabolic profile of each cancer subtype to develop effective therapeutic strategies.
Moreover, the regulation of the Warburg effect is multifaceted, involving oncogenes, tumor suppressor genes, and signaling pathways. Oncogenes such as MYC and RAS can upregulate the expression of glycolytic enzymes and glucose transporters, while tumor suppressor genes such as TP53 can inhibit glycolysis and promote OXPHOS [5]. The PI3K/AKT/mTOR signaling pathway, frequently activated in cancer, plays a critical role in regulating glucose uptake and glycolytic enzyme activity. Hypoxia, a common feature of the tumor microenvironment, activates hypoxia-inducible factor-1 (HIF-1), a transcription factor that induces the expression of glycolytic enzymes and glucose transporters, further promoting the Warburg effect [6].
The concept of the ‘reverse Warburg effect’ has also emerged, describing a scenario where cancer-associated fibroblasts (CAFs) in the tumor stroma undergo aerobic glycolysis and release metabolites, such as lactate and pyruvate, that are then taken up by cancer cells to fuel OXPHOS [7]. This metabolic symbiosis highlights the complex interactions between cancer cells and their surrounding microenvironment and further complicates the understanding of energy metabolism in tumors.
Many thanks to our sponsor Esdebe who helped us prepare this research report.
3. Key Glycolytic Enzymes and Their Roles in Cancer
Glycolysis involves a series of enzymatic reactions that sequentially convert glucose into pyruvate. Several key glycolytic enzymes have been implicated in cancer development and progression, including:
- Hexokinase (HK): HK catalyzes the first committed step of glycolysis, phosphorylating glucose to glucose-6-phosphate. HK2, a specific isoform, is often overexpressed in cancer cells and is associated with increased glucose uptake and glycolytic flux. HK2 is also localized to the outer mitochondrial membrane, where it interacts with the voltage-dependent anion channel (VDAC), facilitating ATP supply to the mitochondria and inhibiting apoptosis [8].
- Phosphofructokinase-1 (PFK1): PFK1 catalyzes the rate-limiting step of glycolysis, phosphorylating fructose-6-phosphate to fructose-1,6-bisphosphate. The activity of PFK1 is tightly regulated by various factors, including ATP, AMP, citrate, and fructose-2,6-bisphosphate. PFKFB3 and PFKFB4, bifunctional enzymes that regulate fructose-2,6-bisphosphate levels, are often overexpressed in cancer cells and promote glycolytic flux [9].
- Pyruvate Kinase (PK): PK catalyzes the final step of glycolysis, converting phosphoenolpyruvate (PEP) to pyruvate. Two major isoforms of PK exist: PKM1 and PKM2. PKM2 is predominantly expressed in cancer cells and embryonic tissues. Unlike PKM1, PKM2 can form tetramers or dimers. The dimeric form of PKM2 is less active and allows glycolytic intermediates to be diverted to anabolic pathways. PKM2 is also regulated by post-translational modifications, such as phosphorylation, which can further modulate its activity and promote cancer cell proliferation and survival [10].
- Lactate Dehydrogenase (LDH): LDH catalyzes the conversion of pyruvate to lactate. LDHA, a specific isoform, is frequently overexpressed in cancer cells and is responsible for the increased lactate production observed in the Warburg effect. Lactate production not only generates NAD+ for continued glycolytic flux but also acidifies the tumor microenvironment, contributing to cancer progression [11].
These enzymes are not merely passive participants in glycolysis; they actively contribute to cancer cell survival, proliferation, and metastasis. Targeting these enzymes individually or in combination represents a promising therapeutic strategy.
Many thanks to our sponsor Esdebe who helped us prepare this research report.
4. Lactate Production and the Acidic Tumor Microenvironment
Increased lactate production is a prominent feature of the Warburg effect and plays a crucial role in shaping the tumor microenvironment. The efflux of lactate from cancer cells leads to acidification of the extracellular space, creating a selective advantage for cancer cells and inhibiting the function of immune cells [12].
The acidic tumor microenvironment has several important consequences:
- Angiogenesis: Acidification promotes the release of pro-angiogenic factors, such as vascular endothelial growth factor (VEGF), stimulating the formation of new blood vessels. Angiogenesis provides cancer cells with nutrients and oxygen, supporting their growth and metastasis [13].
- Extracellular Matrix Remodeling: Acidity promotes the activity of matrix metalloproteinases (MMPs), enzymes that degrade the extracellular matrix (ECM). ECM degradation facilitates cancer cell invasion and metastasis [14].
- Immune Suppression: The acidic microenvironment inhibits the activity of immune cells, such as T cells and natural killer (NK) cells, allowing cancer cells to evade immune surveillance [15].
- Drug Resistance: Acidity can reduce the efficacy of certain chemotherapeutic drugs, either by altering their ionization state or by inhibiting their uptake into cancer cells [16].
- Genomic Instability: Chronic exposure to acidic conditions can compromise DNA repair mechanisms, leading to increased genomic instability and promoting cancer evolution [17].
Furthermore, lactate itself acts as a signaling molecule, influencing various cellular processes. Lactate can be taken up by adjacent cells and used as a fuel source for OXPHOS, contributing to metabolic symbiosis within the tumor. Lactate can also activate signaling pathways, such as the PI3K/AKT/mTOR pathway, promoting cancer cell proliferation and survival [18].
Many thanks to our sponsor Esdebe who helped us prepare this research report.
5. Glycolysis and Cancer Hallmarks
Glycolysis plays a critical role in supporting the hallmarks of cancer, including sustained proliferative signaling, evasion of growth suppressors, resisting cell death, enabling replicative immortality, inducing angiogenesis, activating invasion and metastasis, genome instability and mutation, tumor-promoting inflammation, and evading immune destruction [19].
- Sustained Proliferative Signaling: Glycolysis provides the building blocks and energy required for cell growth and proliferation. Intermediates of glycolysis, such as glucose-6-phosphate and ribose-5-phosphate, are shunted into the pentose phosphate pathway (PPP), generating NADPH and precursors for nucleotide synthesis, essential for DNA replication and cell division [20].
- Evasion of Growth Suppressors: Cancer cells often inactivate tumor suppressor genes that normally inhibit cell growth. Glycolysis can contribute to this evasion by providing a metabolic advantage to cells with compromised growth control mechanisms.
- Resisting Cell Death: Glycolysis can protect cancer cells from apoptosis (programmed cell death) by inhibiting the activation of caspase proteases and by promoting the expression of anti-apoptotic proteins. HK2, for example, inhibits apoptosis by binding to VDAC on the outer mitochondrial membrane [8].
- Enabling Replicative Immortality: Cancer cells often reactivate telomerase, an enzyme that maintains telomere length, allowing them to bypass cellular senescence and achieve replicative immortality. Glycolysis can contribute to this process by providing the energy and building blocks required for telomerase activity.
- Inducing Angiogenesis: As discussed earlier, the acidic tumor microenvironment resulting from increased lactate production stimulates angiogenesis, providing cancer cells with nutrients and oxygen [13].
- Activating Invasion and Metastasis: Glycolysis promotes cancer cell invasion and metastasis by increasing the expression of MMPs and by altering cell adhesion properties [14].
- Genome Instability and Mutation: Increased glycolysis and lactate production can compromise DNA repair mechanisms, leading to genomic instability and promoting the accumulation of mutations [17].
- Tumor-Promoting Inflammation: The acidic tumor microenvironment and the release of lactate can trigger an inflammatory response, which can promote cancer progression by recruiting immune cells that secrete growth factors and cytokines [21].
- Evading Immune Destruction: The acidic tumor microenvironment inhibits the activity of immune cells, allowing cancer cells to evade immune surveillance and destruction [15].
Many thanks to our sponsor Esdebe who helped us prepare this research report.
6. Therapeutic Targeting of Glycolysis: Opportunities and Challenges
The central role of glycolysis in cancer metabolism makes it an attractive therapeutic target. Several strategies have been developed to inhibit glycolysis and disrupt cancer cell growth and survival. These strategies include:
- Glucose Transport Inhibitors: Inhibitors of glucose transporters, such as GLUT1, can reduce glucose uptake into cancer cells, depriving them of their primary fuel source. However, these inhibitors can also affect normal cells that rely on glucose, leading to potential side effects [22].
- Hexokinase Inhibitors: Inhibitors of HK, particularly HK2, can block the first committed step of glycolysis and induce apoptosis in cancer cells. 2-Deoxyglucose (2-DG) is a commonly used HK inhibitor, but its clinical efficacy has been limited due to its non-specific effects and the development of resistance [23].
- PFK1 Activators/Inhibitors: Manipulating PFK1 activity is complex given the allosteric regulation. Targeting PFKFB3/4 has shown promise but may have off-target effects. Further work is needed to develop more selective and effective PFK1 modulators [9].
- Pyruvate Kinase M2 (PKM2) Inhibitors: Inhibiting PKM2, especially in its dimeric form, can disrupt glycolytic flux and redirect metabolic intermediates to anabolic pathways. However, the effects of PKM2 inhibition can be context-dependent, and some studies have shown that it can actually promote cancer cell growth in certain situations [10].
- Lactate Dehydrogenase (LDH) Inhibitors: Inhibiting LDH, particularly LDHA, can reduce lactate production and reverse the acidification of the tumor microenvironment. Several LDH inhibitors are currently in clinical development, and some have shown promising results in preclinical studies [11].
- Dichloroacetate (DCA): DCA inhibits pyruvate dehydrogenase kinase (PDK), which phosphorylates and inactivates pyruvate dehydrogenase (PDH), the enzyme that converts pyruvate to acetyl-CoA for entry into the Krebs cycle. By inhibiting PDK, DCA promotes the flux of pyruvate into the mitochondria, increasing OXPHOS and reducing lactate production [24].
While targeting glycolysis holds significant promise, several challenges need to be addressed. First, cancer cells can develop resistance to glycolytic inhibitors by upregulating alternative metabolic pathways, such as OXPHOS. Second, the toxicity of glycolytic inhibitors to normal cells needs to be minimized. Third, the heterogeneity of cancer metabolism requires a personalized approach to therapy, tailoring treatment strategies to the specific metabolic profile of each patient’s tumor [25].
Combination therapies that target both glycolysis and OXPHOS, or that combine glycolytic inhibitors with conventional chemotherapeutic agents or immunotherapies, may be more effective than single-agent therapies. Furthermore, the development of more selective and potent glycolytic inhibitors is crucial for improving the therapeutic efficacy and reducing the side effects of these agents. Finally, metabolic imaging techniques, such as PET-CT with fluorodeoxyglucose (FDG), can be used to monitor the response of tumors to glycolytic inhibitors and to identify patients who are most likely to benefit from this therapy [26].
Many thanks to our sponsor Esdebe who helped us prepare this research report.
7. Conclusion
Glycolysis is a central metabolic pathway that is frequently dysregulated in cancer cells, contributing to their uncontrolled proliferation, survival, and metastasis. The Warburg effect, characterized by increased glucose uptake and lactate production, is a hallmark of cancer metabolism and plays a crucial role in shaping the tumor microenvironment. Targeting glycolytic pathways represents a promising therapeutic strategy, but several challenges need to be addressed, including the development of resistance, the toxicity to normal cells, and the heterogeneity of cancer metabolism. Future research should focus on developing more selective and potent glycolytic inhibitors, optimizing combination therapies, and utilizing metabolic imaging techniques to personalize treatment strategies and improve patient outcomes. Understanding the metabolic plasticity of cancer cells and their adaptation mechanisms will be crucial for developing effective therapies that can overcome resistance and achieve durable responses. Ultimately, targeting glycolysis, in combination with other therapeutic modalities, holds the potential to significantly improve the treatment of cancer.
Many thanks to our sponsor Esdebe who helped us prepare this research report.
References
[1] Warburg, O. (1956). On the origin of cancer cells. Science, 123(3191), 309-314.
[2] Vander Heiden, M. G., Cantley, L. C., & Thompson, C. B. (2009). Understanding the Warburg effect: the metabolic requirements of cell proliferation. Science, 324(5930), 1029-1033.
[3] Koppenol, W. H., Glynn, N., & Ratanasirintrawoot, S. (2011). Warburg effect and mitochondrial metabolism. Mitochondrion, 11(3), 341-348.
[4] Elia, I., & Fendt, S. M. (2016). Metabolic hallmarks of cancer. Trends in Cancer, 2(4), 198-208.
[5] Dang, C. V. (2012). MYC on the path to cancer. Cell, 149(1), 22-35.
[6] Semenza, G. L. (2014). Oxygen sensing, homeostasis and disease. New England Journal of Medicine, 371(6), 537-547.
[7] Pavlides, V., Whitaker-Menezes, D., Castello-Cros, R., Colombo, J. R., Paradiso, V. W., Pestell, R. G., … & Lisanti, M. P. (2009). The reverse Warburg effect: mitochondrial transfer from cancer-associated fibroblasts to cancer cells. Cell Cycle, 8(23), 3984-4001.
[8] Miyamoto, K., Cutts, B. A., Uecker, A., & Schlossberg, R. (2008). The role of hexokinase II in cancer metabolism. American Journal of Pathology, 173(5), 1471-1476.
[9] Chesney, J. (2020). Targeting PFKFB3: A novel approach to cancer therapy. Seminars in Cancer Biology, 64, 42-48.
[10] Christofk, H. R., Vander Heiden, M. G., Harris, R. J., Ramanathan, A., Gerszten, R. E., Wei, R., … & Cantley, L. C. (2008). The M2 splice isoform of pyruvate kinase is important for cancer cell metabolism and tumour growth. Nature, 452(7184), 230-233.
[11] de la Cruz-López, K. G., Castro-Rivera, E. S., Rodríguez-Cruz, V., Otero-Franqui, G., & Vila-Casas, M. (2019). Lactate in the regulation of tumor microenvironment and cancer progression. Frontiers in Oncology, 9, 1143.
[12] Gillies, R. J., Raghunand, N., Garcia-Martin, M. L., & Gatenby, R. A. (2002). pH imaging: a review of principles and applications. Molecular Imaging, 1(2), 130-139.
[13] Gatenby, R. A., & Gillies, R. J. (2004). Why do cancers have high aerobic glycolysis?. Nature Reviews Cancer, 4(11), 891-899.
[14] Webb, B. A., Chimenti, M., Jacobson, M. P., & Barber, D. L. (2011). Dysregulated pH: a perfect storm for cancer progression. Nature Reviews Cancer, 11(9), 671-677.
[15] Fischer, K., Hoffmann, P., Voelkl, S., Meidenbauer, N., Ammer, J., Edinger, M., … & Mackensen, A. (2007). Inhibitory effect of tumor cell-derived lactic acid on human T cells. Blood, 109(9), 3812-3819.
[16] Mahoney, B. P., Raghunand, N., Baggett, B., Reynolds, I. S., Zhao, H., Gatenby, R. A., & Gillies, R. J. (2003). Targeting proton dynamics and metabolism in cancer therapy. Annals of the New York Academy of Sciences, 986(1), 1-12.
[17] Williams, A. C., & Hogg, N. (2017). Hypoxia and metabolic adaptation drive genomic instability in cancer. Cancer & Metabolism, 5(1), 1-11.
[18] San-Millán, I., & Brooks, G. A. (2017). Reexamining cancer metabolism: lactate production is a common result not the cause. Nature Reviews Cancer, 17(12), 738-753.
[19] Hanahan, D., & Weinberg, R. A. (2011). Hallmarks of cancer: the next generation. Cell, 144(5), 646-674.
[20] Lunt, S. Y., & Vander Heiden, M. G. (2011). Aerobic glycolysis: meeting the metabolic requirements of cell proliferation. Annual Review of Cell and Developmental Biology, 27, 441-464.
[21] Mu, X., & Broer, S. (2015). The role of amino acid transporters in cancer. Amino Acids, 47(10), 1955-1971.
[22] Wood, T. E., Dal Monte, M., Jelinek, A., Lisanti, M. P., Pestell, R. G., & Sotgia, F. (2011). The glycolytic inhibitor 2-deoxy-d-glucose selectively targets the Warburg effect and promotes autophagy. Journal of Biological Chemistry, 286(36), 31107-31116.
[23] Maher, J. C., Wangpaichitr, M., Savaraj, N., & Kurtrock, R. (2011). Hypersensitization to chemotherapy by the glycolysis inhibitor 2-deoxy-D-glucose. Leukemia, 25(5), 729-739.
[24] Bonnet, S., Archer, S. L., Allalunis-Turner, J., Haromy, A., Beaulieu, C., Thompson, R., … & Michelakis, E. D. (2007). A mitochondria-K+ channel axis is suppressed in cancer and its normalization promotes apoptosis and inhibits cancer growth. Cancer Cell, 11(1), 37-51.
[25] Jones, R. G., & Thompson, C. B. (2009). Tumor suppressor genes and cell metabolism: a path to cancer therapy. Genes & Development, 23(5), 537-548.
[26] Higashi, T., & Saga, T. (2019). Metabolic imaging of cancer. Seminars in Nuclear Medicine, 49(1), 3-17.
So, if cancer cells are all about that glycolytic life, does that mean we can starve them out with a low-carb, keto diet? Asking for, uh, my pizza-loving tendencies. Is it that simple, or are cancer cells sneakier than a toddler with a marker?
That’s a great question! It’s not quite as simple as starving cancer cells with a keto diet. While some studies show promise, cancer cells are adaptable and can find alternative fuel sources. The research highlights the complexity of cancer metabolism and the need for targeted therapies, so keep enjoying that pizza!
Editor: MedTechNews.Uk
Thank you to our Sponsor Esdebe
Fascinating deep dive! So, if we tweak glycolysis and throw in some targeted therapies, are we talking about cancer cells getting a metabolic makeover or just a temporary wardrobe change? Asking for a friend…in science.
That’s a great analogy! While some changes might be temporary, combining glycolysis modulation with targeted therapies holds promise for a more profound and lasting impact. It’s all about understanding the specific metabolic vulnerabilities to make sure we’re not just giving them a new outfit, but changing their fundamental biology!
Editor: MedTechNews.Uk
Thank you to our Sponsor Esdebe
So, cancer cells are just sweet-toothed fiends hijacking glucose? Does this mean that a sugar tax on tumors might actually be a viable, albeit highly localized, economic policy? I wonder what the compliance rate would be…
That’s a fun and thought-provoking analogy! The idea of a sugar tax on tumors raises some interesting questions about how we might approach cancer treatment from a different perspective. While direct taxation might be a bit far-fetched, it highlights the potential for targeted therapies to disrupt cancer metabolism. Thanks for your comment, it is really nice to see people thinking outside of the box!
Editor: MedTechNews.Uk
Thank you to our Sponsor Esdebe
Given the complexity of metabolic plasticity in cancer, as highlighted in the report, how can we develop diagnostic tools that accurately capture the dynamic metabolic profiles of tumors in real-time, informing more personalized and adaptive treatment strategies?
That’s a critical question! Real-time monitoring is definitely the holy grail. Advances in biosensors and microfluidic technologies, coupled with sophisticated data analytics, are paving the way for non-invasive or minimally invasive approaches to track these dynamic metabolic shifts. The hope is to create treatments that respond in real time to any cellular changes. Great comment!
Editor: MedTechNews.Uk
Thank you to our Sponsor Esdebe
Given the metabolic plasticity described, what mechanisms enable cancer cells to switch between glycolysis and OXPHOS, and how can therapeutic strategies be designed to target these adaptive transitions, preventing cancer cells from simply shifting their metabolic dependencies?