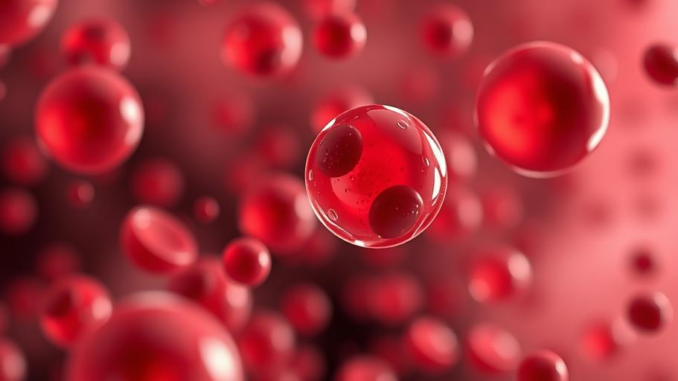
Abstract
Hemoglobin, the primary oxygen-carrying molecule in erythrocytes, is essential for vertebrate life. This report provides a comprehensive overview of hemoglobin, encompassing its intricate structure, functional properties, regulatory mechanisms, and the pathophysiology of hemoglobinopathies. We delve into the molecular details of hemoglobin synthesis, the allosteric regulation of oxygen binding, and the diverse array of genetic mutations that can lead to hemoglobin disorders. Furthermore, we explore the clinical manifestations of these disorders, including sickle cell disease, thalassemia, and other less common hemoglobin variants. Finally, we discuss recent advancements in understanding the molecular mechanisms underlying these diseases and emerging therapeutic strategies aimed at alleviating their symptoms and improving patient outcomes.
Many thanks to our sponsor Esdebe who helped us prepare this research report.
1. Introduction
Hemoglobin (Hb) is a tetrameric protein found in red blood cells (erythrocytes) responsible for transporting oxygen from the lungs to the tissues and carbon dioxide from the tissues back to the lungs. Its efficient oxygen transport is crucial for cellular respiration and overall organismal function. The hemoglobin molecule is composed of four globin subunits, each containing a heme group, which is a porphyrin ring complex with a central iron atom. The iron atom reversibly binds to oxygen, enabling the efficient uptake and delivery of this essential gas. Understanding the structure, function, and regulation of hemoglobin is fundamental to comprehending the physiology of oxygen transport and the pathophysiology of various hematological disorders known as hemoglobinopathies. Hemoglobinopathies arise from genetic mutations affecting either the structure or the synthesis of globin chains, leading to a wide spectrum of clinical manifestations, ranging from mild anemia to severe, life-threatening conditions.
Many thanks to our sponsor Esdebe who helped us prepare this research report.
2. Structure and Assembly of Hemoglobin
2.1. Globin Genes and Protein Structure
The human globin genes are organized into two gene clusters located on chromosomes 16 and 11. The α-globin cluster on chromosome 16 contains the α-globin genes (HBA1 and HBA2) and the ζ-globin gene, while the β-globin cluster on chromosome 11 contains the β-globin gene (HBB), the δ-globin gene (HBD), the γ-globin genes (HBG1 and HBG2), and the ε-globin gene. The α- and β-globin genes are the most abundant and are responsible for the production of adult hemoglobin (HbA), which consists of two α-globin and two β-globin subunits (α2β2). During embryonic and fetal development, different globin subunits are expressed, leading to the formation of different hemoglobin variants. Embryonic hemoglobins include Hb Gower 1 (ζ2ε2), Hb Gower 2 (α2ε2), and Hb Portland (ζ2γ2). Fetal hemoglobin (HbF) consists of two α-globin and two γ-globin subunits (α2γ2). The switch from embryonic to fetal hemoglobin production occurs during early gestation, and HbF remains the predominant hemoglobin variant until shortly after birth, when it is gradually replaced by HbA. The δ-globin gene is expressed at low levels and contributes to the minor adult hemoglobin variant HbA2 (α2δ2).
Each globin subunit consists of approximately 141-146 amino acids and folds into a characteristic tertiary structure known as the globin fold. This fold consists of eight α-helices (labeled A through H) connected by short loops. A hydrophobic pocket within the globin fold cradles the heme group. The heme group is a protoporphyrin IX ring complex with a central iron atom in the ferrous (Fe2+) state. The iron atom is coordinated by four nitrogen atoms from the porphyrin ring and a proximal histidine residue (His F8) from the globin subunit. The sixth coordination site of the iron atom is available for reversible binding of oxygen. The distal histidine residue (His E7) is located near the oxygen-binding site and plays a role in stabilizing oxygen binding and preventing the oxidation of iron.
2.2. Quaternary Structure and Allosteric Regulation
Functional hemoglobin is a tetramer composed of two αβ dimers. The αβ dimers are held together by strong hydrophobic interactions, while the dimers are associated through weaker ionic and hydrogen bonds. This quaternary structure allows for cooperative binding of oxygen, a phenomenon in which the binding of oxygen to one subunit increases the affinity of the other subunits for oxygen. This cooperativity is crucial for efficient oxygen uptake in the lungs and oxygen delivery to the tissues.
Hemoglobin exists in two major conformational states: the T (tense) state and the R (relaxed) state. The T state has a low affinity for oxygen, while the R state has a high affinity for oxygen. The binding of oxygen to hemoglobin induces a conformational change from the T state to the R state. This conformational change involves a rotation of the αβ dimers relative to each other, which alters the interactions between the subunits and increases the affinity for oxygen. Several factors can influence the equilibrium between the T and R states, including pH, carbon dioxide, and 2,3-bisphosphoglycerate (2,3-BPG). Decreased pH (increased acidity), increased carbon dioxide concentration, and increased 2,3-BPG concentration shift the equilibrium towards the T state, reducing the affinity for oxygen and promoting oxygen release to the tissues. Conversely, increased pH, decreased carbon dioxide concentration, and decreased 2,3-BPG concentration shift the equilibrium towards the R state, increasing the affinity for oxygen and promoting oxygen uptake in the lungs. This allosteric regulation of hemoglobin by pH, carbon dioxide, and 2,3-BPG ensures that oxygen delivery is optimized to meet the metabolic demands of the tissues.
Many thanks to our sponsor Esdebe who helped us prepare this research report.
3. Hemoglobin Synthesis
Hemoglobin synthesis is a complex process that involves the coordinated production of globin chains and heme. The process occurs primarily in erythroid precursor cells in the bone marrow. Globin chain synthesis is regulated at the transcriptional and translational levels to ensure that equal amounts of α- and β-globin chains are produced. Heme synthesis is also tightly regulated to match the rate of globin chain synthesis. Defects in either globin chain synthesis or heme synthesis can lead to various forms of anemia.
3.1. Globin Chain Synthesis
Globin chain synthesis is initiated by the transcription of globin genes in the nucleus. The resulting mRNA transcripts are then transported to the cytoplasm, where they are translated by ribosomes. The translation of globin mRNA is regulated by several factors, including the availability of iron and heme. Iron is required for the synthesis of heme, which in turn is required for the stability of globin chains. When iron is deficient, the translation of globin mRNA is inhibited, preventing the accumulation of free globin chains, which can be toxic to cells. Heme also directly regulates globin chain synthesis by binding to and activating heme-regulated inhibitor (HRI) kinase. HRI kinase phosphorylates eIF2α, leading to inhibition of translation initiation. This feedback mechanism ensures that globin chain synthesis is coordinated with heme synthesis.
3.2. Heme Synthesis
Heme synthesis is a multi-step process that occurs in both the mitochondria and the cytoplasm. The initial step involves the condensation of succinyl CoA from the Krebs cycle and glycine to form δ-aminolevulinic acid (ALA). This reaction is catalyzed by ALA synthase, which is the rate-limiting enzyme in heme synthesis. ALA is then transported to the cytoplasm, where it is converted to porphobilinogen (PBG). Four molecules of PBG are then assembled to form protoporphyrin, which is transported back to the mitochondria. In the mitochondria, iron is incorporated into protoporphyrin to form heme. The final step is catalyzed by ferrochelatase. Defects in any of the enzymes involved in heme synthesis can lead to various forms of porphyria, a group of genetic disorders characterized by the accumulation of porphyrin precursors.
Many thanks to our sponsor Esdebe who helped us prepare this research report.
4. Hemoglobinopathies: Genetic Basis and Classification
Hemoglobinopathies are a diverse group of genetic disorders caused by mutations in the globin genes. These mutations can affect either the structure or the synthesis of globin chains, leading to a wide spectrum of clinical manifestations. Hemoglobinopathies are among the most common genetic disorders worldwide, particularly in populations with a high prevalence of malaria. The increased frequency of hemoglobinopathies in these populations is thought to be due to the protective effect of some hemoglobin variants against malaria infection.
4.1. Structural Hemoglobin Variants
Structural hemoglobin variants are caused by mutations that alter the amino acid sequence of the globin chains. These mutations can affect the stability, solubility, or oxygen-binding properties of hemoglobin. Some common structural hemoglobin variants include HbS (sickle cell hemoglobin), HbC, and HbE. HbS is caused by a point mutation in the β-globin gene that substitutes valine for glutamic acid at position 6 (β6Glu→Val). This mutation leads to the polymerization of HbS molecules under conditions of low oxygen tension, resulting in the formation of rigid, sickle-shaped red blood cells. HbC is caused by a point mutation in the β-globin gene that substitutes lysine for glutamic acid at position 6 (β6Glu→Lys). HbC is less soluble than HbA and can crystallize within red blood cells, leading to mild hemolytic anemia. HbE is caused by a point mutation in the β-globin gene that substitutes lysine for glutamic acid at position 26 (β26Glu→Lys). HbE is unstable and is produced at reduced levels, leading to mild thalassemia.
4.2. Thalassemia Syndromes
Thalassemia syndromes are caused by mutations that reduce or abolish the synthesis of one or more globin chains. The reduced synthesis of globin chains leads to an imbalance in the ratio of α- and β-globin chains, resulting in ineffective erythropoiesis and hemolytic anemia. Thalassemia syndromes are classified according to the affected globin chain: α-thalassemia is caused by mutations affecting the α-globin genes, while β-thalassemia is caused by mutations affecting the β-globin gene. The severity of thalassemia depends on the number and type of mutated genes.
4.2.1. α-Thalassemia
α-Thalassemia is most commonly caused by deletions of the α-globin genes. Humans normally have four α-globin genes (two on each chromosome 16). The severity of α-thalassemia depends on the number of deleted α-globin genes. Deletion of one α-globin gene (αα/α-) results in a silent carrier state with no clinical symptoms. Deletion of two α-globin genes (αα/– or α-/α-) results in α-thalassemia trait, which is characterized by mild microcytic anemia. Deletion of three α-globin genes (α-/–) results in hemoglobin H (HbH) disease, which is characterized by moderate to severe hemolytic anemia. Deletion of all four α-globin genes (–/–) results in hydrops fetalis, a fatal condition in which the fetus is severely anemic and edematous.
4.2.2. β-Thalassemia
β-Thalassemia is caused by a variety of mutations in the β-globin gene, including point mutations, insertions, and deletions. These mutations can reduce (β+) or abolish (β0) the synthesis of β-globin chains. β-Thalassemia minor (β+/β or β0/β) is characterized by mild microcytic anemia. β-Thalassemia intermedia is characterized by moderate anemia that requires occasional transfusions. β-Thalassemia major (β0/β0 or β+/β+) is characterized by severe anemia that requires regular blood transfusions for survival. Untransfused patients with β-thalassemia major develop severe complications, including iron overload, growth retardation, and skeletal deformities.
4.3. Hereditary Persistence of Fetal Hemoglobin (HPFH)
HPFH is a group of genetic disorders characterized by the continued production of fetal hemoglobin (HbF) in adulthood. HPFH is caused by mutations in the β-globin gene cluster that prevent the normal switch from γ-globin to β-globin production. Individuals with HPFH have elevated levels of HbF, which can compensate for the deficiency of HbA in patients with β-thalassemia or sickle cell disease. HPFH can be caused by deletions of the δ- and β-globin genes or by point mutations in the γ-globin gene promoters.
Many thanks to our sponsor Esdebe who helped us prepare this research report.
5. Clinical Manifestations and Diagnosis of Hemoglobinopathies
The clinical manifestations of hemoglobinopathies are highly variable and depend on the specific genetic mutation, the severity of the globin chain deficiency, and the presence of other modifying factors. Some common clinical features of hemoglobinopathies include anemia, jaundice, splenomegaly, and bone marrow expansion. Patients with sickle cell disease may also experience vaso-occlusive crises, which are characterized by severe pain caused by the blockage of blood vessels by sickled red blood cells. Patients with thalassemia may develop iron overload due to chronic blood transfusions.
The diagnosis of hemoglobinopathies typically involves a combination of hematological and molecular techniques. Hematological tests include complete blood count (CBC), peripheral blood smear, and hemoglobin electrophoresis. CBC can reveal anemia, microcytosis, and hypochromia. Peripheral blood smear can reveal abnormal red blood cell morphology, such as sickle cells, target cells, and basophilic stippling. Hemoglobin electrophoresis can identify abnormal hemoglobin variants and quantify the relative amounts of different hemoglobins. Molecular tests include DNA sequencing and PCR-based assays to identify specific mutations in the globin genes.
Many thanks to our sponsor Esdebe who helped us prepare this research report.
6. Recent Advances in Understanding and Treating Hemoglobinopathies
Significant progress has been made in recent years in understanding the molecular mechanisms underlying hemoglobinopathies and in developing new therapeutic strategies for these disorders. Some key areas of advancement include:
6.1. Gene Therapy
Gene therapy holds great promise for the treatment of hemoglobinopathies. The goal of gene therapy is to correct the underlying genetic defect by introducing a functional copy of the globin gene into the patient’s hematopoietic stem cells. Several gene therapy approaches are currently being investigated, including lentiviral vector-mediated gene transfer and CRISPR-Cas9 gene editing. Lentiviral vector-mediated gene transfer involves using a modified lentivirus to deliver a functional copy of the β-globin gene into the patient’s hematopoietic stem cells. CRISPR-Cas9 gene editing involves using a guide RNA and the Cas9 enzyme to target and correct specific mutations in the globin genes. Early clinical trials of gene therapy for β-thalassemia and sickle cell disease have shown promising results, with many patients achieving transfusion independence and significant improvement in clinical symptoms. However, long-term safety and efficacy of gene therapy remain to be established.
6.2. CRISPR-Cas9 Gene Editing
CRISPR-Cas9 gene editing is a revolutionary technology that allows for precise modification of DNA sequences. This technology has been successfully used to correct mutations in the globin genes in vitro and in vivo. Several CRISPR-Cas9-based therapies are currently being developed for the treatment of hemoglobinopathies. One approach involves editing the β-globin gene to correct the sickle cell mutation. Another approach involves editing the BCL11A enhancer region to disrupt the expression of BCL11A, a transcriptional repressor of γ-globin gene expression. Disruption of BCL11A leads to increased levels of fetal hemoglobin, which can compensate for the deficiency of HbA in patients with β-thalassemia or sickle cell disease.
6.3. Pharmacological Induction of Fetal Hemoglobin
Pharmacological induction of fetal hemoglobin (HbF) is another promising therapeutic strategy for hemoglobinopathies. Increased levels of HbF can ameliorate the symptoms of β-thalassemia and sickle cell disease by diluting the proportion of abnormal hemoglobin. Several drugs have been shown to induce HbF expression, including hydroxyurea, decitabine, and butyrate derivatives. Hydroxyurea is the most widely used HbF inducer and has been shown to reduce the frequency of vaso-occlusive crises in patients with sickle cell disease. However, hydroxyurea is not effective in all patients and can have significant side effects. Decitabine is a DNA methyltransferase inhibitor that has been shown to induce HbF expression in both preclinical and clinical studies. However, decitabine is associated with a risk of myelosuppression. Butyrate derivatives are histone deacetylase inhibitors that have also been shown to induce HbF expression. New and more potent HbF inducers are currently being developed and tested in clinical trials.
6.4. Novel Therapeutic Targets
In addition to gene therapy and HbF induction, several other novel therapeutic targets are being investigated for the treatment of hemoglobinopathies. These include:
- Inhibition of red blood cell sickling: Several compounds are being developed to inhibit the polymerization of HbS molecules and prevent red blood cell sickling.
- Improvement of red blood cell deformability: Compounds are being developed to improve the deformability of red blood cells, allowing them to pass more easily through the microvasculature.
- Reduction of inflammation: Chronic inflammation plays a significant role in the pathogenesis of sickle cell disease. Anti-inflammatory therapies are being investigated to reduce the inflammatory burden in patients with sickle cell disease.
- Targeting iron overload: Iron overload is a major complication of thalassemia. New iron chelators are being developed to improve iron removal and reduce the risk of iron-related organ damage.
Many thanks to our sponsor Esdebe who helped us prepare this research report.
7. Conclusion
Hemoglobinopathies are a complex group of genetic disorders that pose a significant health burden worldwide. Understanding the structure, function, and regulation of hemoglobin is crucial for developing effective therapies for these disorders. Recent advances in gene therapy, CRISPR-Cas9 gene editing, and pharmacological induction of fetal hemoglobin offer promising new approaches for the treatment of hemoglobinopathies. Continued research into the molecular mechanisms underlying these diseases and the development of novel therapeutic targets will be essential for improving the lives of patients with hemoglobinopathies.
Many thanks to our sponsor Esdebe who helped us prepare this research report.
References
- Bunn, H. F., & Forget, B. G. (1986). Hemoglobin: Molecular, genetic and clinical aspects. WB Saunders.
- Weatherall, D. J. (2010). The Thalassemias. In Hematology: Basic Principles and Practice (5th ed., pp. 523-557). Churchill Livingstone.
- Steinberg, M. H. (2008). Sickle cell anemia as a paradigm of human genetic disease. American Journal of Human Genetics, 83(1), 1-10.
- Thein, S. L. (2005). Genetic modifiers of beta-thalassemia. Haematologica, 90(5), 681-691.
- Cavazzana-Calvo, M., Payen, E., et al. (2010). Transfusion independence and reversal of iron overload in β-thalassemia major patients transplanted with gene-corrected autologous hematopoietic stem cells. Nature medicine, 16(6), 729-731.
- Esrick, E. B., Bauer, D. E., et al. (2021). CRISPR-Cas9 gene editing for sickle cell disease. New England Journal of Medicine, 384(3), 275-277.
- Flaherty, K. R., Shewale, J. B., et al. (2022). Gene therapy for β-thalassemia: a review of current strategies and future directions. Frontiers in Genetics, 13, 913033.
- Nathan, D. G., & Orkin, S. H. (1998). Nathan and Oski’s Hematology of Infancy and Childhood. WB Saunders.
- Olivieri, N. F. (1999). The γ-globin gene switch: a molecular approach to hemoglobinopathies. Blood, 93(9), 2927-2931.
- Sankaran, V. G., & Nathan, D. G. (2015). The molecular and cellular basis of hemoglobinopathies. Cold Spring Harbor Perspectives in Medicine, 5(11), a011720.
So, after all that, we’re still stuck with potential “long-term safety” issues with gene therapy? I thought we were past the point of just shifting the problem to another generation. Are we sure this isn’t just a really expensive band-aid?
That’s a very valid point! The question of long-term safety in gene therapy is paramount. Research is constantly evolving to ensure efficacy and minimize potential risks, especially regarding unintended consequences across generations. It’s a fine balance between innovative solutions and responsible application. Thanks for raising such an important consideration.
Editor: MedTechNews.Uk
Thank you to our Sponsor Esdebe
So, basically, if our hemoglobin isn’t up to par, we’re looking at everything from mild anemia to full-blown hydrops fetalis. Guess it’s not just about having enough iron; it’s about making sure the whole globin party is throwing the right shape. Fascinating stuff!
That’s a great way to put it! The “globin party throwing the right shape” is key. It highlights that it’s not *just* about quantity, but also about the quality and proper assembly of these complex molecules. Minor variations can have huge effects on function. Thanks for the insightful comment!
Editor: MedTechNews.Uk
Thank you to our Sponsor Esdebe
The discussion of allosteric regulation highlights the elegant efficiency of hemoglobin. The way pH, carbon dioxide, and 2,3-BPG fine-tune oxygen delivery based on metabolic needs is truly remarkable and vital to understanding how our bodies adapt to different physiological states.
Thank you for your comment! It’s amazing how the body’s systems work together. The allosteric regulation of hemoglobin is definitely an important process. It allows our bodies to adapt to different needs.
Editor: MedTechNews.Uk
Thank you to our Sponsor Esdebe