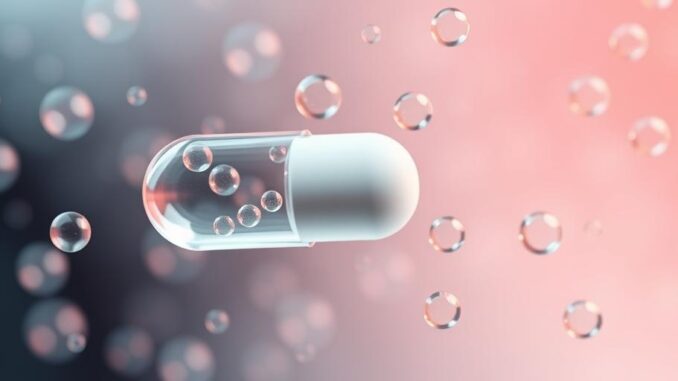
Abstract
Liposomes, self-assembled spherical vesicles composed of lipid bilayers, have emerged as a versatile platform for targeted drug delivery and biomedical research. Their biocompatibility, biodegradability, and ability to encapsulate both hydrophilic and hydrophobic payloads have fueled their widespread adoption. This research report provides a comprehensive overview of liposomes, encompassing their structure, classification, preparation methods, encapsulation techniques, targeting strategies, and diverse applications in drug delivery, diagnostics, and gene therapy. The report further explores the challenges associated with liposome stability, toxicity, and clinical translation, highlighting recent advancements in overcoming these limitations. Furthermore, we will look at the current state of liposomes as delivery vehicles in different contexts and offer some opinions on the direction of travel.
Many thanks to our sponsor Esdebe who helped us prepare this research report.
1. Introduction
Liposomes, initially described by Alec Bangham in the 1960s, are artificially prepared vesicles composed of lipid bilayers surrounding an aqueous core [1]. These structures mimic the biological membranes of cells, rendering them inherently biocompatible and biodegradable. Their unique amphiphilic nature allows for the encapsulation of a wide range of therapeutic agents, including hydrophilic drugs within the aqueous core and hydrophobic drugs within the lipid bilayer. This versatility, coupled with the ability to be tailored for targeted delivery, has made liposomes a prominent tool in drug delivery and biomedical research.
The development of liposomes as drug carriers has traversed several generations, starting with conventional liposomes and progressing to stealth liposomes, targeted liposomes, and stimuli-responsive liposomes [2]. Each generation addresses specific limitations of the previous one, aiming to improve drug encapsulation, circulation time, target specificity, and controlled release. The ongoing research and development in this field are driving the creation of increasingly sophisticated liposomal formulations with enhanced therapeutic efficacy and reduced side effects.
This report aims to provide an in-depth exploration of liposomes, covering their fundamental properties, preparation methodologies, targeting strategies, and applications in various biomedical domains. It also delves into the challenges that hinder their clinical translation and the innovative approaches being developed to overcome these obstacles. The discussion here will attempt to go beyond basic facts and definitions and provide some reasoned opinion.
Many thanks to our sponsor Esdebe who helped us prepare this research report.
2. Structure and Classification of Liposomes
2.1. Liposomal Structure
The fundamental building block of a liposome is the amphiphilic lipid molecule, typically a phospholipid. These molecules possess a hydrophilic (water-attracting) head group and one or two hydrophobic (water-repelling) fatty acid tails. In an aqueous environment, phospholipids spontaneously self-assemble into bilayers, with the hydrophobic tails facing inward and the hydrophilic heads interacting with the surrounding water. This self-assembly process leads to the formation of closed spherical vesicles known as liposomes [3].
2.2. Classification Based on Size, Lamellarity, and Composition
Liposomes can be classified based on several characteristics, including size, lamellarity (number of lipid bilayers), and lipid composition:
- Size: Liposomes range in size from tens of nanometers to several micrometers. They can be broadly categorized as:
- Small Unilamellar Vesicles (SUVs): Size range of 20-100 nm, consisting of a single lipid bilayer.
- Large Unilamellar Vesicles (LUVs): Size range of 100-1000 nm, also consisting of a single lipid bilayer.
- Giant Unilamellar Vesicles (GUVs): Size range of >1 μm, consisting of a single lipid bilayer.
- Multilamellar Vesicles (MLVs): Size range of >100 nm, consisting of multiple concentric lipid bilayers separated by aqueous compartments.
- Lamellarity: The number of lipid bilayers in a liposome determines its lamellarity. Unilamellar liposomes (SUVs, LUVs, and GUVs) have a single bilayer, while multilamellar liposomes (MLVs) have multiple bilayers.
- Composition: The lipid composition of liposomes can be tailored to achieve specific properties. Common lipid components include phospholipids (e.g., phosphatidylcholine, phosphatidylserine), cholesterol, and PEGylated lipids [4]. Cholesterol increases membrane rigidity and reduces permeability, while PEGylation enhances circulation time by preventing opsonization and clearance by the reticuloendothelial system (RES).
Choosing the right lipid composition is essential to optimise the behaviour of liposomes in vivo. For example, saturated lipids produce a more rigid structure and this may be appropriate when increased stability is required. Conversely, unsaturated lipids can improve drug leakage or allow the formation of more flexible and easily deformable liposomes.
Many thanks to our sponsor Esdebe who helped us prepare this research report.
3. Methods of Liposome Preparation
Several methods exist for preparing liposomes, each with its own advantages and disadvantages. The choice of method depends on factors such as the desired size, lamellarity, and encapsulation efficiency [5].
3.1. Thin-Film Hydration Method
This is one of the most widely used methods for preparing MLVs. In this method, lipids are dissolved in an organic solvent (e.g., chloroform, methanol) and evaporated under reduced pressure using a rotary evaporator. This leaves a thin film of lipids on the surface of the flask. The lipid film is then hydrated with an aqueous solution, causing the lipids to self-assemble into MLVs. The resulting MLV suspension can be further processed to obtain SUVs or LUVs using techniques such as sonication or extrusion [6].
3.2. Sonication
Sonication involves exposing a lipid suspension to high-frequency sound waves, which disrupts the lipid bilayers and reduces the size of the liposomes. This method can be used to prepare SUVs from MLVs. However, sonication can also lead to lipid degradation and drug leakage [7].
3.3. Extrusion
Extrusion involves forcing a lipid suspension through a polycarbonate membrane with a defined pore size. This method can be used to prepare liposomes of a specific size and lamellarity. The extrusion process is relatively gentle and minimizes lipid degradation and drug leakage [8].
3.4. Reverse-Phase Evaporation Method
In this method, lipids are dissolved in an organic solvent and emulsified with an aqueous solution containing the drug to be encapsulated. The organic solvent is then evaporated under reduced pressure, leading to the formation of a water-in-oil emulsion. As the solvent evaporates, the lipids self-assemble around the aqueous droplets, forming liposomes with the drug encapsulated in the aqueous core. This method is particularly useful for encapsulating hydrophilic drugs [9].
3.5. Microfluidics
Microfluidic devices offer precise control over the mixing of fluids at the microscale, allowing for the formation of highly uniform liposomes with controlled size and composition. These devices can be used to prepare liposomes with various lipid compositions and encapsulate a wide range of drugs [10].
Microfluidics is a recent development that is gaining traction due to the increased reproducibility of the results. When liposomes are prepared manually there is a significant chance of differences in the final product. One of the biggest challenges in producing liposomes is the batch-to-batch variation and the possibility to reduce this with microfluidics is a big step forward.
Many thanks to our sponsor Esdebe who helped us prepare this research report.
4. Drug Encapsulation Techniques
The ability to efficiently encapsulate therapeutic agents is a key advantage of liposomes. Several techniques can be employed to encapsulate drugs within liposomes, depending on the physicochemical properties of the drug and the desired release profile [11].
4.1. Passive Encapsulation
Passive encapsulation involves incorporating the drug into the liposome during the liposome formation process. This can be achieved by dissolving the drug in the aqueous solution used to hydrate the lipid film in the thin-film hydration method or by adding the drug to the aqueous phase during the reverse-phase evaporation method. Passive encapsulation is a simple and straightforward method, but it often results in low encapsulation efficiency, especially for hydrophilic drugs [12].
4.2. Active Encapsulation
Active encapsulation involves using a transmembrane pH gradient to drive the drug into the liposome. In this method, liposomes are prepared with an acidic internal pH. When the liposomes are incubated with a drug that is a weak base, the drug diffuses across the lipid bilayer in its uncharged form. Once inside the liposome, the drug becomes protonated and charged, preventing it from diffusing back out. This leads to high encapsulation efficiency, especially for weakly basic drugs [13].
4.3. Remote Loading
Remote loading techniques involve encapsulating the drug after the liposomes have been formed. This can be achieved by using ion gradients, such as ammonium sulfate gradients or citrate gradients, to drive the drug into the liposome. Remote loading techniques are particularly useful for encapsulating large or charged molecules that cannot readily cross the lipid bilayer [14].
The selection of the encapsulation technique depends on several factors including the drug, the delivery conditions and the cost. It is not always necessary to have an extremely high percentage of the drug encapsulated if the production costs become too prohibitive. The final choice must be a compromise between all of the factors.
Many thanks to our sponsor Esdebe who helped us prepare this research report.
5. Targeting Strategies
Targeting strategies are crucial for enhancing the therapeutic efficacy of liposomes and reducing off-target effects. By modifying the surface of liposomes with targeting ligands, they can be directed to specific cells or tissues [15].
5.1. Passive Targeting
Passive targeting relies on the inherent properties of liposomes to accumulate in certain tissues. For example, liposomes tend to accumulate in the liver and spleen due to the enhanced permeability and retention (EPR) effect in these organs. The EPR effect is a phenomenon observed in tumors, where the leaky vasculature allows for the extravasation of macromolecules, such as liposomes, into the tumor microenvironment. Liposomes can also passively target inflamed tissues due to the increased vascular permeability in these areas [16].
5.2. Active Targeting
Active targeting involves modifying the surface of liposomes with targeting ligands that bind to specific receptors on target cells. Common targeting ligands include antibodies, antibody fragments, peptides, aptamers, and small molecules. When the liposome encounters a target cell expressing the corresponding receptor, the targeting ligand binds to the receptor, triggering receptor-mediated endocytosis and internalization of the liposome [17].
5.3. Stimuli-Responsive Targeting
Stimuli-responsive liposomes are designed to release their payload in response to specific stimuli present in the target microenvironment. These stimuli can include changes in pH, temperature, redox potential, or enzyme activity. Stimuli-responsive liposomes can be used to achieve targeted drug delivery by releasing the drug only at the site of action, minimizing off-target effects [18]. For example, liposomes can be designed to be stable at physiological pH but to become destabilized and release their payload in the acidic environment of tumors.
Choosing the best targeting strategy requires knowledge of the site of action of the drug. Often, a combination of targeting strategies is employed, in order to ensure maximum delivery and efficiency. The surface of the liposome can be modified to include several functional groups. Care must be taken to ensure that the loading and modifications do not impact the stability of the liposome.
Many thanks to our sponsor Esdebe who helped us prepare this research report.
6. Applications of Liposomes in Drug Delivery and Biomedical Research
Liposomes have found widespread applications in drug delivery and biomedical research due to their versatility and biocompatibility [19].
6.1. Cancer Therapy
Liposomal formulations of anticancer drugs, such as doxorubicin (Doxil®) and paclitaxel (Abraxane®), have been successfully used in cancer therapy. These formulations offer several advantages over conventional formulations, including reduced toxicity, improved drug delivery to tumors, and enhanced therapeutic efficacy [20]. Liposomes can also be used to deliver other anticancer agents, such as siRNA and chemotherapeutic prodrugs.
6.2. Vaccine Delivery
Liposomes have been explored as vaccine delivery vehicles due to their ability to encapsulate antigens and adjuvants and to stimulate both humoral and cellular immune responses. Liposomal vaccines have shown promise in protecting against various infectious diseases, including influenza, HIV, and malaria [21].
6.3. Gene Therapy
Liposomes can be used to deliver genes or oligonucleotides to target cells. Cationic liposomes are often used for gene delivery because they can complex with negatively charged DNA or RNA molecules. Liposomal gene therapy has shown promise in treating various genetic disorders and cancers [22].
6.4. Diagnostic Imaging
Liposomes can be used as contrast agents for diagnostic imaging techniques, such as MRI, CT, and ultrasound. By encapsulating imaging agents within liposomes, their biodistribution and accumulation in target tissues can be controlled, leading to improved image quality and diagnostic accuracy [23].
6.5. Cosmetic Applications
Liposomes are widely used in cosmetics and personal care products to deliver active ingredients to the skin. Liposomal formulations can improve the penetration of active ingredients through the stratum corneum and enhance their efficacy [24].
Liposomes have seen a huge increase in use in recent years. The ability to formulate liposomes with a wide variety of therapeutic agents has made them very attractive. This is a rapidly expanding area and the future of liposomes is promising.
Many thanks to our sponsor Esdebe who helped us prepare this research report.
7. Challenges and Future Directions
Despite their numerous advantages, liposomes face several challenges that hinder their clinical translation [25].
7.1. Stability
Liposomes can be unstable in vivo, undergoing aggregation, fusion, and drug leakage. Strategies to improve liposome stability include incorporating cholesterol into the lipid bilayer, coating the liposomes with polymers such as PEG, and lyophilizing the liposomes [26].
7.2. Toxicity
Some liposomal formulations can be toxic, especially those containing cationic lipids or high concentrations of certain lipids. The toxicity of liposomes depends on their composition, size, charge, and route of administration. Strategies to reduce liposome toxicity include using biocompatible lipids, optimizing the lipid composition, and modifying the surface of the liposomes with polymers [27].
7.3. Clinical Translation
The clinical translation of liposomal formulations can be challenging due to factors such as regulatory hurdles, manufacturing complexities, and cost considerations. Efforts are underway to develop more efficient and scalable manufacturing processes for liposomes and to streamline the regulatory approval process [28].
7.4. Future Directions
Future research directions in the field of liposomes include:
- Developing more sophisticated targeting strategies to improve drug delivery to specific cells or tissues.
- Developing stimuli-responsive liposomes that can release their payload in response to specific triggers in the target microenvironment.
- Developing liposomes that can cross biological barriers, such as the blood-brain barrier.
- Developing liposomes that can deliver multiple therapeutic agents simultaneously.
- Developing liposomes that can be used for theranostic applications, combining diagnosis and therapy.
One interesting area of development is the use of artificial intelligence (AI) to optimise the formulation and design of liposomes. There are so many parameters that can be tweaked in the design of a liposome that AI may provide a faster and more efficient mechanism to develop new treatments.
Many thanks to our sponsor Esdebe who helped us prepare this research report.
8. Conclusion
Liposomes have emerged as a powerful platform for targeted drug delivery and biomedical research. Their biocompatibility, biodegradability, and versatility have made them attractive for a wide range of applications, including cancer therapy, vaccine delivery, gene therapy, diagnostic imaging, and cosmetics. While liposomes face several challenges related to stability, toxicity, and clinical translation, ongoing research and development efforts are focused on overcoming these limitations and expanding the clinical utility of liposomes. The future of liposomes is promising, with the potential to revolutionize the treatment of various diseases and improve human health.
Many thanks to our sponsor Esdebe who helped us prepare this research report.
References
[1] Bangham, A. D., Standish, M. M., & Watkins, J. C. (1965). Diffusion of univalent ions across the lamellae of swollen phospholipids. Journal of Molecular Biology, 13(1), 238-252.
[2] Allen, T. M., & Cullis, P. R. (2004). Liposomal drug delivery systems: from concept to clinical applications. Advanced Drug Delivery Reviews, 56(5), 491-501.
[3] Lasic, D. D. (1993). Liposomes: from physics to applications. Elsevier.
[4] Sercombe, L., Veerati, T., Wu, J., Zhou, D., Kirkbride, P., Bryant, J., & Porter, C. J. (2015). Advances and challenges of liposome assisted drug delivery. Frontiers in Pharmacology, 6, 286.
[5] Akbarzadeh, A., Rezaei-Sadabady, R., Davaran, S., Joo, S. W., Zarghami, N., Hanifehpour, Y., Samiei, M., Khorramizadeh, H. R., & Mehrabi, M. R. (2013). Liposome: classification, preparation, and applications. Nanoscale Research Letters, 8(1), 102.
[6] Szoka, F. C., Jr., & Papahadjopoulos, D. (1978). Procedure for preparation of liposomes with large internal aqueous space and high capture by reverse-phase evaporation. Proceedings of the National Academy of Sciences, 75(9), 4194-4198.
[7] Hope, M. J., Bally, M. B., Webb, G., & Cullis, P. R. (1985). Production of large unilamellar vesicles by a rapid extrusion procedure. Biochimica et Biophysica Acta (BBA)-Biomembranes, 812(1), 55-65.
[8] Mayer, L. D., Hope, M. J., Cullis, P. R., & Janoff, A. S. (1986). Vesicles of variable sizes produced by a rapid extrusion technique. Biochimica et Biophysica Acta (BBA)-Biomembranes, 858(2), 161-168.
[9] Gregoriadis, G. (1993). Liposome technology. CRC press.
[10] Hood, R. R., DeForest, C. A., & Lewis, L. H. (2021). High-throughput microfluidic synthesis of liposomes for drug delivery. Advanced Drug Delivery Reviews, 177, 113935.
[11] Allen, T. M., & Cullis, P. R. (2013). Liposomal drug delivery systems: from concept to clinical applications. Advanced Drug Delivery Reviews, 65(1), 36-48.
[12] Vemuri, S., & Rhodes, C. T. (1995). Preparation and characterization of liposomes as vehicles for oral delivery of proteins. Pharmaceutical Technology, 19(10), 44-52.
[13] Maurer, N., Fenske, D. B., Cullis, P. R., & Dykstra, K. (2001). Development of stable and efficient encapsulation methods for anticancer drugs in liposomes. Journal of Controlled Release, 76(1-2), 159-169.
[14] Madden, T. D., Harrigan, P. R., Tai, L. C. L., Bally, M. B., Mayer, L. D., Redelmeier, T. E., Loughrey, H. C., & Hope, M. J. (1990). The accumulation of drugs within large unilamellar vesicles exhibiting a proton gradient. Chemistry and Physics of Lipids, 53(1), 23-30.
[15] Peer, D., Karp, J. M., Hong, S., Farokhzad, O. C., Margalit, R., & Langer, R. (2007). Nanocarriers for targeted cancer therapy. Nature Nanotechnology, 2(12), 751-760.
[16] Matsumura, Y., & Maeda, H. (1986). A new concept for macromolecular therapeutics in cancer chemotherapy: mechanism of tumoritropic accumulation of proteins and the antitumor agent smancs. Cancer Research, 46(12 Pt 1), 6387-6392.
[17] Sapra, P., & Allen, T. M. (2003). Improved outcome when B16LS melanoma-bearing mice are treated with targeted liposomal doxorubicin in combination with anti-CD40 antibody. Clinical Cancer Research, 9(17), 6243-6255.
[18] Mura, S., Nicolas, J., & Couvreur, P. (2013). Stimuli-responsive nanocarriers for drug delivery. Nature Materials, 12(11), 991-1003.
[19] Torchilin, V. P. (2005). Recent advances with liposomes as pharmaceutical carriers. Nature Reviews Drug Discovery, 4(2), 145-160.
[20] Barenholz, Y. (2012). Doxil®—the first FDA-approved nano-drug: lessons learned. Journal of Controlled Release, 160(2), 117-134.
[21] Manjili, M. H., Park, J., Fesnak, A., Dao, T., Raziuddin, A., Barnett, D., … & Lee, C. P. (2003). Induction of potent antitumor immunity with targeted and nontargeted liposomes containing mannosylated tumor-associated carbohydrate antigens. Cancer Immunology, Immunotherapy, 52(2), 96-105.
[22] Felgner, P. L., Gadek, T. R., Holm, M., Roman, R., Chan, H. W., Wenz, M., Northrop, J. P., Ringold, G. M., & Danielsen, M. (1987). Lipofection: a highly efficient, lipid-mediated DNA-transfection procedure. Proceedings of the National Academy of Sciences, 84(21), 7413-7417.
[23] Bulte, J. W., Kraitchman, D. L., Bottomley, P. A., & Gupta, A. (2002). In vivo USPIO labeling of macrophages in rat liver. Radiology, 223(3), 615-623.
[24] Cevc, G., & Blume, G. (1992). Lipid vesicles penetrate into intact skin owing to the transdermal osmotic gradients and hydration force. Biochimica et Biophysica Acta (BBA)-Biomembranes, 1104(1), 156-166.
[25] Immordino, M. L., Dosio, F., & Cattel, L. (2006). Stealth liposomes: review of the basic science, rationale, and clinical applications, existing and potential. International Journal of Nanomedicine, 1(3), 297.
[26] Gabizon, A., & Papahadjopoulos, D. (1988). Liposomes in drug delivery. Basic Mechanisms of Antitumor Drug Resistance. Springer, Boston, MA, 415-426.
[27] Hofheinz, R. D., Gnad-Vogt, S. U., Beyer, U., & Ricke, A. (2005). Liposomal encapsulated drugs. Drug Discover Today, 10(9), 583-596.
[28] Paillard, A., Häffner, K., Montanari, J., Schneider, M., & Stébé, M. J. (2010). Liposome scale-up: from laboratory to industrial production. European Journal of Pharmaceutics and Biopharmaceutics, 76(3), 412-430.
Given the challenges in liposome stability, could you expand on the latest advancements in lipid chemistry or surface modifications that show promise in extending circulation time and reducing premature drug release in vivo?
That’s a great point! Extending circulation time is key. Beyond PEGylation, researchers are exploring novel lipids like bio-degradable phospholipids and incorporating unique polymers into the bilayer structure. These can offer controlled drug release and increased stability, leading to more effective targeted therapies. It’s a rapidly evolving field!
Editor: MedTechNews.Uk
Thank you to our Sponsor Esdebe
So, if AI’s calling the shots on liposome design now, does that mean we’ll soon have personalized liposomes tailored to our individual genetic quirks? Or will we just end up with a bunch of super-optimized but eerily similar nanobots?
That’s a fascinating question! The potential for AI to personalize liposome design based on individual genetics is definitely on the horizon. While standardization might offer efficiency, the true power lies in tailoring treatments for optimal efficacy. It will be interesting to observe these advances in the near future.
Editor: MedTechNews.Uk
Thank you to our Sponsor Esdebe
Given the potential of AI in liposome design, how might we address concerns regarding data privacy and algorithmic transparency to ensure responsible development and equitable access to these advanced therapies?
That’s a crucial question! Focusing on data privacy and transparency is essential as we integrate AI into liposome design. Perhaps federated learning approaches, where models are trained on decentralized data without direct access, could be a viable path forward. This could ensure responsible development. What do you think?
Editor: MedTechNews.Uk
Thank you to our Sponsor Esdebe
AI optimizing liposome design… intriguing! But with so many variables already, is there a risk AI might get stuck in a local optimum, churning out liposomes that are *good enough*, but miss that truly groundbreaking formulation? Or are we safe from nano-mediocrity?
That’s a fantastic question! The risk of AI settling for “good enough” solutions is definitely something to consider. Perhaps incorporating evolutionary algorithms or reinforcement learning could help the AI explore a broader range of possibilities and escape those local optima. What strategies do you think could be most effective?
Editor: MedTechNews.Uk
Thank you to our Sponsor Esdebe
AI optimizing liposome design, eh? I’m all for efficiency, but let’s hope the robots don’t start demanding royalties for their nano-creations. Will we need tiny AI ethicists for our tiny drug carriers?