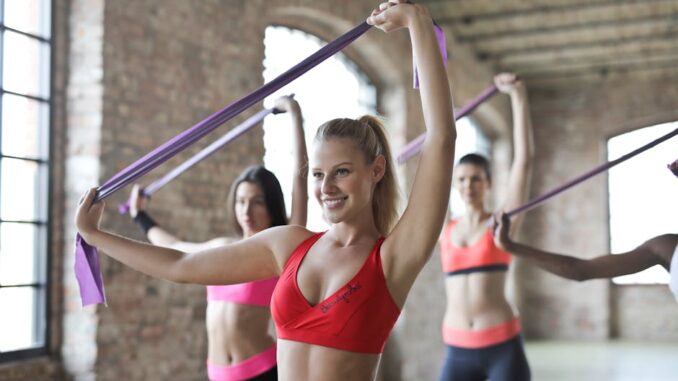
Abstract
Metabolic flexibility, the capacity of an organism to efficiently switch between fuel sources depending on availability and demand, is increasingly recognized as a critical determinant of metabolic health. Impaired metabolic flexibility is implicated in a wide range of pathologies, including type 2 diabetes, cardiovascular disease, neurodegenerative disorders, and even cancer. This report explores the multifaceted nature of metabolic flexibility, encompassing the underlying molecular mechanisms, the factors influencing its regulation (including diet, exercise, and aging), and its clinical significance. We delve into the cellular processes governing substrate oxidation, mitochondrial function, and hormonal regulation, examining how these contribute to the overall metabolic flexibility of an organism. Furthermore, we critically evaluate current methods for assessing metabolic flexibility and discuss potential therapeutic strategies aimed at restoring or enhancing metabolic flexibility to improve health outcomes. Finally, we highlight the research gaps and future directions in this rapidly evolving field.
Many thanks to our sponsor Esdebe who helped us prepare this research report.
1. Introduction
The human body is a complex biochemical machine constantly adapting to fluctuating energy demands and nutrient availability. A hallmark of metabolic health is the ability to efficiently transition between different fuel sources – primarily carbohydrates and fats – to maintain energy homeostasis. This adaptability, termed metabolic flexibility, allows the body to respond appropriately to changes in diet, exercise, and physiological state. In metabolically healthy individuals, insulin promotes glucose uptake and oxidation during periods of carbohydrate abundance, while during fasting or exercise, the body readily switches to fat oxidation to conserve glucose for glucose-dependent tissues like the brain. Impaired metabolic flexibility, conversely, signifies an inability to efficiently switch between fuel sources, leading to metabolic inflexibility. This inflexibility is characterized by persistent reliance on glucose oxidation even when fatty acids are readily available or an inability to appropriately suppress glucose oxidation during fasting, resulting in metabolic dysfunction and disease.
This report aims to provide a comprehensive overview of metabolic flexibility, covering its physiological importance, underlying mechanisms, factors influencing its regulation, clinical implications, and potential therapeutic targets. We will also address the challenges associated with measuring metabolic flexibility and highlight areas that require further research.
Many thanks to our sponsor Esdebe who helped us prepare this research report.
2. Defining Metabolic Flexibility
Metabolic flexibility, at its core, reflects the capacity of an organism to adapt fuel utilization to prevailing metabolic demands. It is not simply about the ability to oxidize both carbohydrates and fats, but rather the appropriate and efficient shift between these fuel sources in response to physiological cues. This adaptability is crucial for maintaining glucose homeostasis, preventing the accumulation of ectopic lipids, and ensuring adequate energy supply to tissues.
Several aspects contribute to metabolic flexibility:
- Substrate Switching: The ability to readily transition between glucose and fatty acid oxidation based on substrate availability and energy requirements.
- Insulin Sensitivity: The responsiveness of tissues to insulin, allowing for efficient glucose uptake and utilization following carbohydrate ingestion.
- Mitochondrial Function: The capacity of mitochondria to efficiently oxidize both glucose and fatty acids, and to adjust oxidative capacity to meet energy demands.
- Hormonal Regulation: The coordinated action of hormones, such as insulin, glucagon, and epinephrine, to regulate fuel metabolism.
- Tissue-Specific Adaptations: The ability of different tissues (e.g., muscle, liver, adipose tissue) to adapt their metabolic profile to meet their specific energy needs and contribute to overall metabolic homeostasis. Muscle, for example, plays a significant role in glucose disposal and fat oxidation during exercise, while the liver regulates glucose production and lipid metabolism.
Failure in any of these areas can contribute to metabolic inflexibility, resulting in impaired glucose tolerance, insulin resistance, and an increased risk of metabolic diseases.
Many thanks to our sponsor Esdebe who helped us prepare this research report.
3. Mechanisms Underlying Metabolic Flexibility
Metabolic flexibility is governed by a complex interplay of cellular and molecular mechanisms, including:
3.1. Regulation of Substrate Oxidation
The primary determinant of metabolic flexibility is the regulation of substrate oxidation, specifically the balance between glucose and fatty acid oxidation. This balance is controlled by several key enzymes and regulatory pathways:
- Glucose Transport: Insulin stimulates glucose uptake in muscle and adipose tissue via the translocation of GLUT4 glucose transporters to the cell membrane. Impaired GLUT4 translocation is a hallmark of insulin resistance and contributes to reduced glucose oxidation.
- Glycolysis: The rate of glycolysis, the pathway for glucose breakdown, is regulated by enzymes such as hexokinase, phosphofructokinase, and pyruvate kinase. Insulin stimulates glycolysis, while fatty acid oxidation can inhibit glycolysis through the accumulation of citrate, an inhibitor of phosphofructokinase.
- Fatty Acid Uptake: Fatty acid uptake is mediated by fatty acid translocase (FAT/CD36) and fatty acid transport proteins (FATPs). Insulin and muscle contraction can increase fatty acid uptake, while hormones like epinephrine promote lipolysis and fatty acid release from adipose tissue.
- Fatty Acid Oxidation (FAO): FAO occurs in the mitochondria and is regulated by carnitine palmitoyltransferase 1 (CPT1), which controls the entry of fatty acids into the mitochondria. Malonyl-CoA, an intermediate in fatty acid synthesis, inhibits CPT1, providing a mechanism for reciprocal regulation of glucose and fatty acid oxidation. During periods of carbohydrate abundance, elevated malonyl-CoA inhibits FAO, promoting glucose oxidation. Conversely, during fasting or exercise, low malonyl-CoA allows for increased FAO.
- Pyruvate Dehydrogenase (PDH): PDH is a key enzyme that links glycolysis to the tricarboxylic acid (TCA) cycle and is a critical point of regulation in glucose oxidation. PDH activity is inhibited by phosphorylation by pyruvate dehydrogenase kinase (PDK) and stimulated by dephosphorylation by pyruvate dehydrogenase phosphatase (PDP). Insulin promotes PDH activation, while fatty acid oxidation increases PDK expression, inhibiting PDH and diverting pyruvate away from oxidation.
3.2. Mitochondrial Function
Mitochondria are the powerhouses of the cell, responsible for ATP production through oxidative phosphorylation. Mitochondrial function is critical for metabolic flexibility, as it determines the capacity of the cell to efficiently oxidize both glucose and fatty acids. Key aspects of mitochondrial function relevant to metabolic flexibility include:
- Mitochondrial Biogenesis: The process of creating new mitochondria. Exercise and caloric restriction can stimulate mitochondrial biogenesis, increasing mitochondrial capacity and improving metabolic flexibility.
- Mitochondrial Dynamics: The processes of mitochondrial fusion and fission, which are important for maintaining mitochondrial health and function. Imbalances in mitochondrial dynamics are associated with metabolic dysfunction.
- Respiratory Chain Function: The efficiency of the electron transport chain in generating ATP. Impaired respiratory chain function can lead to reduced oxidative capacity and metabolic inflexibility.
- Mitochondrial Uncoupling: The process of dissipating the proton gradient across the mitochondrial membrane, leading to increased heat production and reduced ATP production. Mild uncoupling can improve metabolic flexibility by increasing substrate flux through the TCA cycle and increasing fatty acid oxidation. UCP1 in brown adipose tissue is a key regulator of non-shivering thermogenesis and contributes to energy expenditure.
3.3. Hormonal Regulation
Hormones play a crucial role in regulating metabolic flexibility by coordinating fuel metabolism in response to physiological cues. Key hormones involved in metabolic flexibility include:
- Insulin: Insulin is the primary anabolic hormone that promotes glucose uptake, glycolysis, and glycogen synthesis. It also inhibits lipolysis and promotes fatty acid synthesis. Insulin resistance, characterized by a reduced response to insulin, is a major contributor to metabolic inflexibility.
- Glucagon: Glucagon is a catabolic hormone that stimulates glycogenolysis, gluconeogenesis, and lipolysis. It opposes the actions of insulin and is important for maintaining glucose homeostasis during fasting.
- Epinephrine: Epinephrine, released during stress or exercise, stimulates glycogenolysis and lipolysis, providing fuel for increased energy demands. It also increases heart rate and blood pressure.
- Cortisol: Cortisol, a glucocorticoid hormone, promotes gluconeogenesis and lipolysis. Chronic elevation of cortisol is associated with insulin resistance and metabolic dysfunction.
- Adipokines: Adipose tissue secretes a variety of hormones, called adipokines, that regulate metabolism. Adiponectin, for example, improves insulin sensitivity and promotes fatty acid oxidation, while leptin regulates appetite and energy expenditure. Dysregulation of adipokine secretion is associated with obesity and metabolic inflexibility.
3.4. Cellular Signaling Pathways
Numerous cellular signaling pathways contribute to metabolic flexibility. These pathways regulate gene expression, protein synthesis, and enzyme activity, ultimately influencing substrate oxidation and mitochondrial function. Some key pathways include:
- AMPK (AMP-activated protein kinase): AMPK is a cellular energy sensor that is activated by low energy states (e.g., during exercise or caloric restriction). AMPK activation stimulates glucose uptake, fatty acid oxidation, and mitochondrial biogenesis, improving metabolic flexibility.
- mTOR (mammalian target of rapamycin): mTOR is a nutrient sensor that regulates cell growth, proliferation, and metabolism. mTOR activation promotes protein synthesis and inhibits autophagy, while mTOR inhibition promotes autophagy and improves insulin sensitivity.
- PPARs (peroxisome proliferator-activated receptors): PPARs are a family of nuclear receptors that regulate gene expression involved in lipid metabolism, glucose metabolism, and inflammation. PPARα promotes fatty acid oxidation, while PPARγ promotes adipogenesis and insulin sensitivity.
- SIRT1 (sirtuin 1): SIRT1 is a NAD+-dependent deacetylase that regulates gene expression and protein activity involved in glucose metabolism, lipid metabolism, and mitochondrial function. SIRT1 activation is associated with improved metabolic health and lifespan extension.
Many thanks to our sponsor Esdebe who helped us prepare this research report.
4. Factors Influencing Metabolic Flexibility
Metabolic flexibility is influenced by a variety of factors, including:
4.1. Diet
The composition and timing of meals significantly impact metabolic flexibility. Diets high in processed carbohydrates and saturated fats can impair insulin sensitivity and reduce the ability to efficiently switch between fuel sources. Conversely, diets rich in whole foods, fiber, and healthy fats can improve metabolic flexibility. Intermittent fasting and time-restricted feeding have gained increasing attention for their potential to enhance metabolic flexibility by promoting substrate switching and improving insulin sensitivity. The timing of nutrient intake also plays a crucial role. Consuming the majority of calories earlier in the day may align better with circadian rhythms and improve metabolic outcomes.
4.2. Exercise
Regular exercise is a potent stimulus for improving metabolic flexibility. Exercise increases glucose uptake, fatty acid oxidation, and mitochondrial biogenesis. Both aerobic and resistance training can improve metabolic flexibility, although different types of exercise may have distinct effects on fuel metabolism. Aerobic exercise primarily increases fatty acid oxidation capacity, while resistance training can improve glucose disposal and muscle mass.
4.3. Aging
Metabolic flexibility declines with age, contributing to an increased risk of metabolic diseases. Age-related changes in muscle mass, mitochondrial function, and hormonal regulation contribute to this decline. Sarcopenia (loss of muscle mass) reduces glucose disposal capacity, while age-related mitochondrial dysfunction impairs oxidative capacity. Hormonal changes, such as decreased growth hormone and testosterone levels, can also contribute to metabolic inflexibility.
4.4. Genetics
Genetic factors also play a role in determining metabolic flexibility. Variations in genes involved in glucose metabolism, lipid metabolism, and mitochondrial function can influence an individual’s susceptibility to metabolic diseases. Epigenetic modifications, such as DNA methylation and histone modifications, can also influence gene expression and metabolic flexibility.
4.5. Gut Microbiota
The gut microbiota plays a crucial role in regulating metabolism and influencing metabolic flexibility. Gut bacteria can ferment dietary fiber into short-chain fatty acids (SCFAs), which are absorbed into the bloodstream and used as fuel by the host. SCFAs can also influence glucose metabolism, lipid metabolism, and inflammation. Dysbiosis, an imbalance in the gut microbiota, is associated with metabolic dysfunction and impaired metabolic flexibility.
4.6. Sleep
Adequate sleep is essential for metabolic health. Sleep deprivation can impair glucose tolerance, reduce insulin sensitivity, and increase cortisol levels, all of which contribute to metabolic inflexibility. Maintaining a regular sleep schedule and optimizing sleep quality are important for promoting metabolic flexibility.
4.7. Environmental Toxins
Exposure to environmental toxins, such as endocrine-disrupting chemicals (EDCs), can disrupt metabolic homeostasis and impair metabolic flexibility. EDCs can interfere with hormone signaling, alter gene expression, and disrupt mitochondrial function. Minimizing exposure to environmental toxins is important for maintaining metabolic health.
Many thanks to our sponsor Esdebe who helped us prepare this research report.
5. Clinical Significance of Metabolic Flexibility
Impaired metabolic flexibility is a hallmark of metabolic diseases, including:
5.1. Type 2 Diabetes
Type 2 diabetes is characterized by insulin resistance and impaired glucose tolerance. Individuals with type 2 diabetes exhibit reduced glucose uptake, impaired suppression of hepatic glucose production, and an inability to efficiently switch between glucose and fatty acid oxidation. Metabolic inflexibility is a major contributor to the development and progression of type 2 diabetes.
5.2. Cardiovascular Disease
Metabolic inflexibility is associated with an increased risk of cardiovascular disease. Impaired fatty acid oxidation and ectopic lipid accumulation contribute to atherosclerosis and endothelial dysfunction. Insulin resistance and hyperinsulinemia also promote inflammation and increase the risk of cardiovascular events.
5.3. Non-Alcoholic Fatty Liver Disease (NAFLD)
NAFLD is characterized by the accumulation of fat in the liver in the absence of excessive alcohol consumption. Metabolic inflexibility is a key factor in the pathogenesis of NAFLD. Impaired fatty acid oxidation and increased lipogenesis contribute to the accumulation of fat in the liver. Insulin resistance also promotes hepatic steatosis.
5.4. Neurodegenerative Diseases
Emerging evidence suggests that metabolic inflexibility may play a role in the development of neurodegenerative diseases, such as Alzheimer’s disease and Parkinson’s disease. The brain relies heavily on glucose for energy, and impaired glucose metabolism can lead to neuronal dysfunction and cell death. Insulin resistance in the brain may also contribute to cognitive decline.
5.5. Cancer
Cancer cells often exhibit altered metabolism, characterized by increased glucose uptake and glycolysis, even in the presence of oxygen (the Warburg effect). Metabolic inflexibility may contribute to the survival and proliferation of cancer cells. Targeting cancer cell metabolism is an emerging therapeutic strategy.
Many thanks to our sponsor Esdebe who helped us prepare this research report.
6. Assessment of Metabolic Flexibility
Assessing metabolic flexibility can be challenging, as it requires measuring the ability to efficiently switch between fuel sources in response to physiological cues. Several methods are used to assess metabolic flexibility:
6.1. Respiratory Quotient (RQ)
The RQ is the ratio of carbon dioxide production to oxygen consumption (VCO2/VO2) and provides an estimate of substrate oxidation. An RQ of 1 indicates primarily glucose oxidation, while an RQ of 0.7 indicates primarily fatty acid oxidation. Measuring RQ during different metabolic states (e.g., fasting, exercise, glucose challenge) can provide an indication of metabolic flexibility. A larger change in RQ in response to a change in metabolic state indicates better metabolic flexibility.
6.2. Oral Glucose Tolerance Test (OGTT)
The OGTT measures glucose tolerance and insulin sensitivity. It involves measuring blood glucose and insulin levels before and after consuming a glucose load. Impaired glucose tolerance and insulin resistance are indicative of metabolic inflexibility.
6.3. Insulin Clamp
The insulin clamp is a more sophisticated method for assessing insulin sensitivity. It involves infusing insulin at a constant rate to maintain a steady-state insulin level and measuring the amount of glucose required to maintain a constant blood glucose level. The glucose infusion rate is a measure of insulin sensitivity.
6.4. Hyperinsulinemic-Euglycemic Clamp with Indirect Calorimetry
This technique combines the insulin clamp with indirect calorimetry, allowing for the simultaneous measurement of insulin sensitivity and substrate oxidation rates. This provides a comprehensive assessment of metabolic flexibility.
6.5. Metabolomics
Metabolomics involves measuring the levels of metabolites in biological samples (e.g., blood, urine, tissue) to identify metabolic signatures associated with metabolic flexibility or inflexibility. This approach can provide insights into the underlying metabolic pathways that are dysregulated in metabolic diseases.
6.6. Molecular Markers
Several molecular markers can be used to assess metabolic flexibility, including the expression of genes involved in glucose metabolism, lipid metabolism, and mitochondrial function. Measuring the activity of enzymes involved in substrate oxidation can also provide an indication of metabolic flexibility.
Many thanks to our sponsor Esdebe who helped us prepare this research report.
7. Therapeutic Strategies to Enhance Metabolic Flexibility
Given the importance of metabolic flexibility for health, there is growing interest in developing therapeutic strategies to enhance it. Potential strategies include:
7.1. Dietary Interventions
- Low-Carbohydrate Diets: Low-carbohydrate diets, such as the ketogenic diet, can improve metabolic flexibility by promoting fatty acid oxidation and reducing reliance on glucose. However, these diets may not be suitable for everyone and require careful monitoring.
- Intermittent Fasting: Intermittent fasting involves alternating periods of eating and fasting. It can improve metabolic flexibility by promoting substrate switching, improving insulin sensitivity, and stimulating autophagy.
- Time-Restricted Feeding: Time-restricted feeding involves limiting food intake to a specific window of time each day. It can improve metabolic flexibility by aligning food intake with circadian rhythms and promoting substrate switching.
- Mediterranean Diet: The Mediterranean diet, rich in whole foods, fruits, vegetables, and healthy fats, can improve metabolic flexibility by promoting insulin sensitivity and reducing inflammation.
7.2. Exercise Training
- Aerobic Exercise: Aerobic exercise increases fatty acid oxidation capacity and improves insulin sensitivity.
- Resistance Training: Resistance training increases muscle mass and improves glucose disposal.
- High-Intensity Interval Training (HIIT): HIIT involves short bursts of intense exercise followed by periods of rest. It can improve both aerobic and anaerobic fitness and may be particularly effective for improving metabolic flexibility.
7.3. Pharmacological Interventions
- Metformin: Metformin is a commonly used drug for treating type 2 diabetes. It improves insulin sensitivity and reduces hepatic glucose production.
- Thiazolidinediones (TZDs): TZDs are a class of drugs that improve insulin sensitivity by activating PPARγ. However, they have several side effects, including weight gain and fluid retention.
- SGLT2 Inhibitors: SGLT2 inhibitors are a class of drugs that lower blood glucose by inhibiting glucose reabsorption in the kidneys. They can also improve insulin sensitivity and promote weight loss.
- AMPK Activators: AMPK activators stimulate glucose uptake, fatty acid oxidation, and mitochondrial biogenesis. They are being investigated as potential therapeutic agents for metabolic diseases.
- SIRT1 Activators: SIRT1 activators improve glucose metabolism, lipid metabolism, and mitochondrial function. Resveratrol is a naturally occurring SIRT1 activator.
7.4. Modulation of the Gut Microbiota
- Probiotics: Probiotics are live microorganisms that can improve gut health and influence metabolism. Certain probiotic strains may improve insulin sensitivity and promote weight loss.
- Prebiotics: Prebiotics are non-digestible fibers that promote the growth of beneficial gut bacteria. They can improve gut health and influence metabolism.
- Fecal Microbiota Transplantation (FMT): FMT involves transferring fecal bacteria from a healthy donor to a recipient. It can restore gut microbial diversity and improve metabolic health.
Many thanks to our sponsor Esdebe who helped us prepare this research report.
8. Future Directions and Research Gaps
While significant progress has been made in understanding metabolic flexibility, several research gaps remain:
- Standardization of Assessment Methods: There is a need for standardized methods for assessing metabolic flexibility to allow for better comparison of results across studies.
- Longitudinal Studies: Longitudinal studies are needed to examine the long-term effects of interventions on metabolic flexibility and health outcomes.
- Personalized Approaches: Personalized approaches are needed to identify the optimal strategies for improving metabolic flexibility in different individuals, taking into account their genetics, lifestyle, and gut microbiota.
- Tissue-Specific Metabolic Flexibility: More research is needed to understand tissue-specific metabolic flexibility and how different tissues contribute to overall metabolic homeostasis.
- Role of Environmental Factors: Further research is needed to investigate the role of environmental factors, such as toxins and pollutants, on metabolic flexibility.
- Mechanistic Studies: More mechanistic studies are needed to elucidate the molecular mechanisms underlying metabolic flexibility and the effects of different interventions.
Many thanks to our sponsor Esdebe who helped us prepare this research report.
9. Conclusion
Metabolic flexibility is a critical determinant of metabolic health and plays a crucial role in preventing and treating metabolic diseases. Improving metabolic flexibility through dietary interventions, exercise training, pharmacological interventions, and modulation of the gut microbiota holds great promise for improving health outcomes and extending lifespan. Future research should focus on addressing the remaining research gaps and developing personalized strategies to enhance metabolic flexibility.
Many thanks to our sponsor Esdebe who helped us prepare this research report.
References
- Galgani, J. E., et al. “Metabolic flexibility and insulin resistance.” American Journal of Physiology-Endocrinology and Metabolism 295.6 (2008): E1009-E1017.
- Holloszy, J. O. “Exercise-induced increase in muscle insulin sensitivity.” Journal of Applied Physiology 99.1 (2005): 338-343.
- Kelley, D. E., et al. “Fuel selection and insulin resistance: a comparison of glucose versus lipid oxidation.” Diabetes 42.12 (1993): 1766-1776.
- Mueller, M. J., et al. “Metabolic flexibility as an adaptation to energy surplus and deficit in humans.” American Journal of Clinical Nutrition 101.6 (2015): 1125-1135.
- Patti, M. E., and D. E. Kahn. “The cellular basis of insulin resistance.” The Journal of Clinical Investigation 108.5 (2001): 573-581.
- Smith, R. L., et al. “Metabolic flexibility: a new therapeutic target for the treatment of metabolic syndrome.” Journal of Translational Medicine 11.1 (2013): 178.
- Goodpaster, B. H., et al. “The effect of weight loss on skeletal muscle lipid metabolism in obese subjects.” Diabetes 49.12 (2000): 2191-2197.
- Zurlo, F., et al. “Skeletal muscle metabolism is a major determinant of resting energy expenditure.” Journal of Clinical Investigation 86.5 (1990): 1423-1427.
- DeFronzo, R. A., et al. “Insulin resistance: a multifaceted syndrome responsible for NIDDM, obesity, hypertension, dyslipidemia, and atherosclerotic cardiovascular disease.” Diabetes Care 14.3 (1991): 173-194.
- Bergman, R. N. “Minimal model: perspective from 2020.” Diabetes 69.5 (2020): 851-863.
- Hawley, J. A., and L. J. Hargreaves. “Skeletal muscle fuel selection and fatigue during prolonged exercise.” Journal of Applied Physiology 77.2 (1994): 614-618.
- Lustig, R. H. “Fructose 2.0: metabolic, genetic, and societal implications of fructose excess.” American Journal of Clinical Nutrition 91.6 (2010): 1387-1394.
- Gill, S., et al. “Time-restricted feeding improves metabolic health and hepatic steatosis in mice fed a high-fat diet.” Cell Metabolism 22.5 (2015): 789-798.
- Longo, V. D., and M. P. Mattson. “Fasting: molecular mechanisms and clinical applications.” Cell Metabolism 19.2 (2014): 181-192.
- Valenzuela, C. A., et al. “Exercise reverses age-associated declines in skeletal muscle mitochondrial function.” PLoS One 10.1 (2015): e0115717.
- Dillin, A., et al. “Rates of behavior and aging modulated by mitochondrial function and AMPK.” Science 305.5690 (2004): 1590-1593.
- Handschin, C., and B. M. Spiegelman. “PGC-1alpha and exercise: a molecular transducer for adaptive physiology.” Annals of the New York Academy of Sciences 1126 (2008): 23-31.
- Musi, N., et al. “AMPK-mediated mechanisms for the beneficial effects of exercise on glucose homeostasis.” Diabetes 50.11 (2001): 2506-2511.
- Cantó, C., and J. Auwerx. “PGC-1alpha switching in metabolism, cancer, and aging.” Trends in Endocrinology & Metabolism 20.10 (2009): 455-464.
- Turnbaugh, P. J., et al. “An obesity-associated gut microbiome with increased capacity for energy harvest.” Nature 444.7122 (2006): 1027-1031.
- Thaiss, C. A., et al. “Microbiota DiGeSting the Host: Implications for Metabolic Diseases.” Cell 163.3 (2015): 507-517.
- Scheer, F. A. J. L., et al. “Adverse metabolic and cardiovascular consequences of circadian misalignment.” Proceedings of the National Academy of Sciences 106.11 (2009): 4453-4458.
- Caspersen, C. J., et al. “Physical activity, exercise, and physical fitness: definitions and distinctions for health-related research.” Public Health Reports 100.2 (1985): 126.
- Hotamisligil, G. S. “Inflammation and metabolic disease.” Nature 462.7278 (2006): 491-497.
- Saltiel, A. R., and C. R. Kahn. “Insulin signalling and the regulation of glucose and lipid metabolism.” Nature 414.6865 (2001): 799-806.
- Newgard, C. B. “Metabolic inflexibility in disease.” Cell Metabolism 15.3 (2012): 259-266.
- Rabol, R., et al. “Impact of a Low Carbohydrate Diet on Glycemic Control, Insulin Sensitivity and Skeletal Muscle Fuel Metabolism in Type 2 Diabetes: Results from a Randomized Clinical Trial.” Nutrients 13.10 (2021): 3581.
“So, the report suggests improving metabolic flexibility could extend lifespan. Does that mean we’re one mitochondria-boosting supplement away from immortality, or are there more gym sessions in my future?”
That’s a great question! While some supplements may offer support, our research points to exercise, especially when combined with dietary adjustments, as having a more significant impact on metabolic flexibility and overall health. It’s a marathon, not a sprint!
Editor: MedTechNews.Uk
Thank you to our Sponsor Esdebe