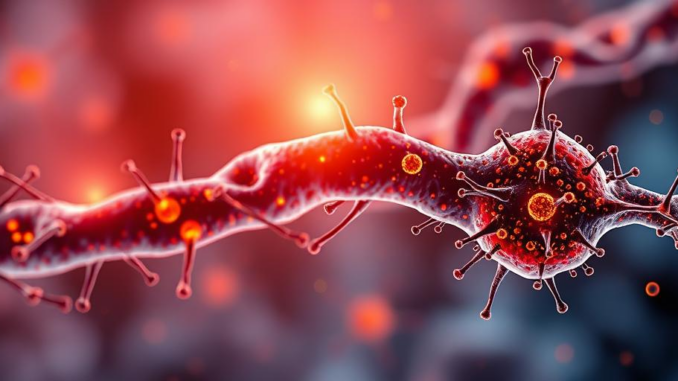
Abstract
Mitochondria, the powerhouses of the cell, play a critical role in cellular energy production, calcium homeostasis, apoptosis, and numerous other vital processes. Mounting evidence implicates mitochondrial dysfunction as a key player in the pathogenesis of a wide spectrum of metabolic diseases, including diabetes, obesity, cardiovascular disease, and neurodegenerative disorders. This review explores the multifaceted roles of mitochondria in metabolic health and disease, focusing on the underlying mechanisms of mitochondrial dysfunction, including impairments in oxidative phosphorylation, mitochondrial dynamics, mitophagy, and mitochondrial DNA (mtDNA) integrity. Furthermore, we examine the latest research on therapeutic strategies targeting mitochondria to prevent or treat metabolic diseases, encompassing pharmacological interventions, lifestyle modifications, and emerging approaches such as gene therapy and stem cell transplantation. Finally, we delve into current methods used to assess mitochondrial function, from in vitro assays to in vivo imaging techniques, highlighting their strengths and limitations.
Many thanks to our sponsor Esdebe who helped us prepare this research report.
1. Introduction
Mitochondria, eukaryotic cell organelles descended from endosymbiotic alpha-proteobacteria, are essential for cellular life. Their primary function is to generate adenosine triphosphate (ATP) through oxidative phosphorylation (OXPHOS), utilizing electrons derived from the oxidation of glucose, fatty acids, and amino acids. However, mitochondria are far more than just ATP factories. They are central hubs for numerous metabolic pathways, including the Krebs cycle, fatty acid oxidation, and amino acid metabolism. Furthermore, they participate in crucial cellular processes such as calcium signaling, reactive oxygen species (ROS) production, apoptosis, and inflammation. The interconnectedness of these functions highlights the importance of maintaining mitochondrial integrity and functionality for overall cellular and organismal health.
Mitochondrial dysfunction, characterized by impaired ATP production, increased ROS generation, altered calcium homeostasis, and disrupted signaling pathways, has been increasingly recognized as a critical factor in the development and progression of various metabolic diseases. The link between mitochondrial dysfunction and metabolic disease is bidirectional. Metabolic stress, such as hyperglycemia, hyperlipidemia, and oxidative stress, can directly damage mitochondria, leading to further impairment of their function. Conversely, primary mitochondrial defects can disrupt metabolic homeostasis, increasing susceptibility to metabolic diseases. This creates a vicious cycle that exacerbates both mitochondrial and metabolic dysfunction.
This review aims to provide a comprehensive overview of the role of mitochondria in metabolic health and disease. We will explore the specific mechanisms of mitochondrial dysfunction, discuss the impact of mitochondrial dysfunction on various metabolic diseases, and review the latest advances in therapeutic strategies targeting mitochondria. Furthermore, we will examine the available methodologies for testing mitochondrial function, including their specific applications and limitations.
Many thanks to our sponsor Esdebe who helped us prepare this research report.
2. Mechanisms of Mitochondrial Dysfunction
Mitochondrial dysfunction is a complex phenomenon resulting from the interplay of various factors. These factors can be broadly categorized as:
2.1 Impaired Oxidative Phosphorylation (OXPHOS)
OXPHOS, the primary ATP-generating pathway in mitochondria, involves the transfer of electrons through a series of protein complexes (Complexes I-IV) in the inner mitochondrial membrane, coupled with the pumping of protons across the membrane to create an electrochemical gradient. This gradient drives ATP synthase (Complex V) to synthesize ATP. Impairment in any of these complexes can reduce ATP production and increase electron leakage, leading to ROS generation.
Several mechanisms can contribute to OXPHOS dysfunction. Genetic mutations in mtDNA, which encodes for 13 essential subunits of the OXPHOS complexes, are a common cause of mitochondrial disorders. These mutations can affect the assembly, stability, or activity of the OXPHOS complexes. In addition, defects in the assembly factors required for proper complex formation can also lead to OXPHOS dysfunction. Furthermore, post-translational modifications, such as phosphorylation and acetylation, can regulate the activity of OXPHOS complexes and can be altered in metabolic disease. For example, increased phosphorylation of pyruvate dehydrogenase kinase (PDK) in diabetes inhibits the activity of pyruvate dehydrogenase (PDH), the enzyme that converts pyruvate to acetyl-CoA, thereby reducing the entry of glucose-derived carbon into the Krebs cycle and OXPHOS.
2.2 Mitochondrial Dynamics
Mitochondria are highly dynamic organelles that constantly undergo fusion and fission, processes collectively known as mitochondrial dynamics. Fusion promotes the mixing of mitochondrial contents, allowing for the complementation of damaged components and the maintenance of mitochondrial function. Fission is essential for mitochondrial division during cell division, for the removal of damaged mitochondria through mitophagy, and for the regulation of mitochondrial distribution within the cell. Imbalances in mitochondrial dynamics have been implicated in various metabolic diseases.
Key regulators of mitochondrial fusion include mitofusin 1 (MFN1), mitofusin 2 (MFN2), and optic atrophy 1 (OPA1). MFN1 and MFN2 are GTPases located on the outer mitochondrial membrane, while OPA1 is a dynamin-related GTPase located on the inner mitochondrial membrane. Mutations in these genes can cause severe mitochondrial disorders. In metabolic diseases, alterations in the expression or activity of these proteins can disrupt mitochondrial fusion. For example, reduced MFN2 expression has been observed in insulin-resistant tissues, contributing to impaired mitochondrial function. Fission is mediated by dynamin-related protein 1 (DRP1), a cytosolic protein that is recruited to the outer mitochondrial membrane and constricts the mitochondrion, leading to its division. Increased DRP1 activity or recruitment to mitochondria has been observed in several metabolic diseases, promoting excessive mitochondrial fragmentation.
2.3 Mitophagy
Mitophagy is a selective form of autophagy that removes damaged or dysfunctional mitochondria. This process is crucial for maintaining mitochondrial quality control and preventing the accumulation of dysfunctional mitochondria, which can trigger inflammation and cell death. Mitophagy is mediated by various proteins, including PTEN-induced kinase 1 (PINK1) and Parkin. PINK1 accumulates on the outer mitochondrial membrane of damaged mitochondria and recruits Parkin, an E3 ubiquitin ligase, to the mitochondria. Parkin ubiquitinates mitochondrial proteins, targeting the mitochondria for degradation by autophagy.
Impaired mitophagy has been implicated in the pathogenesis of several metabolic diseases. For example, reduced mitophagy in pancreatic beta cells has been shown to contribute to beta cell dysfunction and apoptosis in diabetes. In contrast, increased mitophagy has been observed in certain contexts, such as in response to prolonged exercise, and may represent an adaptive response to remove damaged mitochondria and promote mitochondrial biogenesis. Thus, the role of mitophagy in metabolic disease is complex and context-dependent.
2.4 Mitochondrial DNA (mtDNA) Mutations
mtDNA, a small circular genome located within mitochondria, encodes for 13 essential subunits of the OXPHOS complexes, as well as transfer RNAs and ribosomal RNAs required for mitochondrial protein synthesis. mtDNA is highly susceptible to mutations due to its proximity to ROS generated during OXPHOS, its limited DNA repair capacity, and the lack of protective histones. mtDNA mutations can be either inherited or acquired and can range from single nucleotide polymorphisms (SNPs) to large-scale deletions. These mutations can disrupt mitochondrial protein synthesis, impair OXPHOS, and increase ROS generation.
Several mtDNA mutations have been associated with metabolic diseases. For example, the m.3243A>G mutation in the tRNALeu(UUR) gene is a common cause of mitochondrial encephalomyopathy, lactic acidosis, and stroke-like episodes (MELAS), a mitochondrial disorder characterized by neurological dysfunction, muscle weakness, and metabolic abnormalities. Acquired mtDNA mutations can accumulate with age and have been implicated in age-related metabolic diseases, such as diabetes and sarcopenia.
2.5 ROS Production and Oxidative Stress
Mitochondria are a major source of ROS, such as superoxide anion and hydrogen peroxide. ROS are produced as a byproduct of electron transport in the OXPHOS complexes, particularly at Complexes I and III. While ROS play important roles in cellular signaling and regulation, excessive ROS production can lead to oxidative stress, a state of imbalance between ROS production and antioxidant defense mechanisms. Oxidative stress can damage mitochondrial proteins, lipids, and DNA, further impairing mitochondrial function. Furthermore, ROS can activate inflammatory signaling pathways, contributing to chronic inflammation.
In metabolic diseases, increased glucose and fatty acid metabolism can overwhelm the capacity of the OXPHOS system, leading to increased electron leakage and ROS production. Furthermore, impaired antioxidant defenses, such as reduced expression of superoxide dismutase (SOD) and glutathione peroxidase (GPx), can exacerbate oxidative stress. Oxidative stress is a major contributor to the pathogenesis of diabetes, obesity, and cardiovascular disease.
2.6 Calcium Homeostasis
Mitochondria play a critical role in regulating intracellular calcium homeostasis. They can rapidly take up calcium from the cytosol, buffering calcium transients and preventing excessive calcium accumulation. Mitochondrial calcium uptake is mediated by the mitochondrial calcium uniporter (MCU), a protein complex located on the inner mitochondrial membrane. Calcium influx into mitochondria regulates several metabolic enzymes, including PDH and isocitrate dehydrogenase, thereby influencing ATP production. Furthermore, calcium signaling is essential for regulating mitochondrial dynamics, mitophagy, and apoptosis.
In metabolic diseases, altered calcium homeostasis can disrupt mitochondrial function. For example, prolonged exposure to high glucose can lead to increased calcium influx into mitochondria, causing mitochondrial overload and dysfunction. Furthermore, impaired calcium signaling can disrupt mitochondrial dynamics and mitophagy, leading to the accumulation of damaged mitochondria.
Many thanks to our sponsor Esdebe who helped us prepare this research report.
3. Mitochondrial Dysfunction in Specific Metabolic Diseases
3.1 Diabetes Mellitus
In diabetes, mitochondrial dysfunction is implicated in both insulin resistance and beta-cell dysfunction. In insulin-resistant tissues, such as skeletal muscle and adipose tissue, mitochondrial dysfunction impairs fatty acid oxidation, leading to the accumulation of lipid intermediates, such as diacylglycerol (DAG) and ceramide. These lipid intermediates activate protein kinase C (PKC) isoforms, which interfere with insulin signaling and reduce glucose uptake. Furthermore, mitochondrial dysfunction increases ROS production, leading to oxidative stress and inflammation, which further contribute to insulin resistance.
In pancreatic beta cells, mitochondrial dysfunction impairs glucose-stimulated insulin secretion (GSIS). Beta cells rely on mitochondrial ATP production to trigger insulin release. Impaired OXPHOS reduces ATP production, blunting the GSIS response. Furthermore, mitochondrial dysfunction increases ROS production, leading to beta-cell apoptosis and reduced beta-cell mass. Genetic variants affecting mitochondrial function can increase susceptibility to type 2 diabetes. Moreover, exposure to glucotoxic and lipotoxic conditions exacerbates mitochondrial dysfunction in beta cells, contributing to the progressive decline in beta-cell function observed in diabetes.
3.2 Obesity
Obesity is characterized by excessive accumulation of adipose tissue, often associated with inflammation and metabolic dysfunction. Mitochondrial dysfunction in adipose tissue is a key contributor to obesity-related metabolic complications. In obese individuals, adipocytes exhibit impaired mitochondrial function, including reduced OXPHOS capacity and increased ROS production. This leads to reduced fatty acid oxidation and increased lipid storage, further exacerbating adipose tissue dysfunction. Furthermore, mitochondrial dysfunction in adipose tissue promotes inflammation, contributing to systemic insulin resistance.
In addition, mitochondrial dysfunction in other tissues, such as skeletal muscle and liver, also contributes to the pathogenesis of obesity. Impaired mitochondrial function in skeletal muscle reduces energy expenditure and promotes fat accumulation. In the liver, mitochondrial dysfunction contributes to non-alcoholic fatty liver disease (NAFLD), characterized by lipid accumulation and inflammation in the liver.
3.3 Cardiovascular Disease
Mitochondrial dysfunction is a significant factor in the development and progression of cardiovascular diseases, including heart failure, atherosclerosis, and hypertension. In cardiomyocytes, mitochondrial dysfunction impairs ATP production, leading to contractile dysfunction and heart failure. Furthermore, mitochondrial dysfunction increases ROS production, leading to oxidative stress and cardiomyocyte damage. Impaired mitochondrial dynamics and mitophagy contribute to the accumulation of damaged mitochondria in cardiomyocytes.
In vascular endothelial cells, mitochondrial dysfunction impairs nitric oxide (NO) production, a crucial vasodilator. Reduced NO production contributes to endothelial dysfunction, a hallmark of atherosclerosis. Furthermore, mitochondrial dysfunction increases ROS production, promoting inflammation and plaque formation in arteries. In hypertension, mitochondrial dysfunction in vascular smooth muscle cells contributes to increased vascular tone and elevated blood pressure.
3.4 Neurodegenerative Diseases
Mitochondrial dysfunction is a prominent feature of many neurodegenerative diseases, including Alzheimer’s disease, Parkinson’s disease, and Huntington’s disease. Neurons are highly dependent on mitochondrial ATP production to maintain their function. Mitochondrial dysfunction impairs neuronal energy metabolism, leading to synaptic dysfunction, neuronal damage, and ultimately, neuronal death. Furthermore, mitochondrial dysfunction increases ROS production, leading to oxidative stress and neuronal damage. Impaired mitochondrial dynamics and mitophagy contribute to the accumulation of damaged mitochondria in neurons.
In Alzheimer’s disease, amyloid-beta plaques and tau tangles disrupt mitochondrial function and promote oxidative stress. In Parkinson’s disease, mutations in genes involved in mitochondrial dynamics (e.g., Parkin, PINK1) and mitophagy contribute to mitochondrial dysfunction and neuronal loss. In Huntington’s disease, the mutant huntingtin protein disrupts mitochondrial function and promotes oxidative stress.
Many thanks to our sponsor Esdebe who helped us prepare this research report.
4. Therapeutic Strategies Targeting Mitochondria
Given the central role of mitochondrial dysfunction in metabolic diseases, therapeutic strategies targeting mitochondria have gained increasing attention. These strategies can be broadly categorized as:
4.1 Pharmacological Interventions
Several pharmacological agents have been developed to target mitochondria and improve their function. These include:
- Mitochondrial antioxidants: Compounds such as MitoQ and SkQ1 are targeted to mitochondria and scavenge ROS, reducing oxidative stress. MitoQ has shown promise in improving insulin sensitivity and reducing cardiovascular risk factors in some studies.
- Mitochondrial biogenesis enhancers: Peroxisome proliferator-activated receptor gamma coactivator 1-alpha (PGC-1α) activators, such as bezafibrate, stimulate mitochondrial biogenesis, increasing mitochondrial mass and improving mitochondrial function.
- Mitochondrial uncouplers: Low doses of mitochondrial uncouplers, such as 2,4-dinitrophenol (DNP), can slightly reduce the proton gradient across the inner mitochondrial membrane, increasing energy expenditure and reducing ROS production. However, DNP is highly toxic and has limited clinical use. Safer uncouplers, such as BAM15, are being developed.
- Mitochondrial division inhibitor 1 (Mdivi-1): Inhibits DRP1 mediated mitochondrial fission. Mdivi-1 prevents excessive mitochondrial fragmentation and protects against mitochondrial dysfunction.
- Elamipretide (Bendavia): Elamipretide is a small peptide that binds to cardiolipin in the inner mitochondrial membrane, improving mitochondrial structure and function. Elamipretide has shown promise in treating heart failure and other mitochondrial disorders.
4.2 Lifestyle Modifications
Lifestyle modifications, such as exercise and caloric restriction, can also improve mitochondrial function. Exercise stimulates mitochondrial biogenesis, increasing mitochondrial mass and improving OXPHOS capacity. Furthermore, exercise increases antioxidant defenses and reduces oxidative stress. Caloric restriction reduces metabolic stress and promotes mitochondrial autophagy, removing damaged mitochondria and improving mitochondrial quality.
4.3 Novel Approaches
Emerging approaches for treating mitochondrial dysfunction include:
- Gene therapy: Gene therapy aims to correct genetic defects in mtDNA or nuclear genes encoding mitochondrial proteins. Adeno-associated virus (AAV) vectors are commonly used to deliver genes into cells. Gene therapy has shown promise in treating mitochondrial disorders in preclinical studies.
- Stem cell transplantation: Stem cells, such as mesenchymal stem cells (MSCs), can differentiate into various cell types and can be used to replace damaged cells with healthy cells. Furthermore, MSCs can secrete factors that promote mitochondrial biogenesis and improve mitochondrial function.
- Mitochondrial transplantation: Transferring healthy mitochondria into cells with dysfunctional mitochondria can restore mitochondrial function. This approach has shown promise in treating ischemia-reperfusion injury and other mitochondrial disorders. The technique is technically challenging and faces obstacles regarding the host immune response.
- mtDNA editing: Using CRISPR/Cas systems to correct mtDNA mutations is a promising but challenging approach. The delivery of CRISPR/Cas systems into mitochondria is technically difficult, and off-target effects are a concern.
Many thanks to our sponsor Esdebe who helped us prepare this research report.
5. Methods for Testing Mitochondrial Function
Assessing mitochondrial function is crucial for understanding the role of mitochondria in metabolic health and disease and for evaluating the efficacy of therapeutic interventions. Various methods are available for testing mitochondrial function, ranging from in vitro assays to in vivo imaging techniques. Each method has its strengths and limitations.
5.1 In Vitro Assays
- Oxygen consumption rate (OCR): OCR measures the rate at which cells or isolated mitochondria consume oxygen, providing an indication of OXPHOS activity. Seahorse XF analyzers are commonly used to measure OCR in real-time. By adding specific inhibitors of the OXPHOS complexes, the contributions of different complexes to respiration can be assessed.
- ATP production: ATP levels can be measured using various methods, including luciferase-based assays and high-performance liquid chromatography (HPLC). ATP levels provide a direct measure of cellular energy production.
- ROS production: ROS production can be measured using fluorescent probes, such as dichlorofluorescein (DCF) and MitoSOX Red. These probes react with ROS, producing a fluorescent signal that can be quantified using a plate reader or flow cytometry.
- Mitochondrial membrane potential (ΔΨm): ΔΨm is the electrochemical gradient across the inner mitochondrial membrane, which is essential for ATP production. ΔΨm can be measured using fluorescent dyes, such as tetramethylrhodamine ethyl ester (TMRE) and rhodamine 123. These dyes accumulate in mitochondria based on their charge, and changes in ΔΨm can be detected as changes in fluorescence intensity.
- Mitochondrial enzyme activity: The activity of specific mitochondrial enzymes, such as citrate synthase, succinate dehydrogenase, and cytochrome c oxidase, can be measured using spectrophotometric assays. These assays provide information about the function of individual mitochondrial enzymes.
- Mitochondrial DNA copy number: mtDNA copy number can be measured using quantitative polymerase chain reaction (qPCR). Changes in mtDNA copy number can indicate mitochondrial biogenesis or mitophagy.
5.2 In Vivo Imaging Techniques
- Magnetic resonance spectroscopy (MRS): MRS can be used to measure the levels of metabolites, such as ATP and phosphocreatine (PCr), in vivo. This provides information about cellular energy metabolism in different tissues.
- Positron emission tomography (PET): PET can be used to image mitochondrial function in vivo. For example, the tracer [18F]DPA-714 can be used to image mitochondrial translocator protein (TSPO), a marker of mitochondrial inflammation.
- Two-photon microscopy: Two-photon microscopy can be used to image mitochondrial structure and function in vivo. For example, the fluorescent dye rhodamine 123 can be used to measure mitochondrial membrane potential in live cells.
5.3 Limitations of Current Methods
While these methods provide valuable information about mitochondrial function, they also have limitations. In vitro assays are performed in artificial conditions that may not accurately reflect the in vivo environment. In vivo imaging techniques are often limited by their spatial resolution and sensitivity. Furthermore, most methods only assess a limited number of mitochondrial parameters, while mitochondrial function is a complex and multifaceted process. Therefore, it is important to use a combination of methods to assess mitochondrial function comprehensively.
Many thanks to our sponsor Esdebe who helped us prepare this research report.
6. Conclusion
Mitochondrial dysfunction is a central mediator in the pathogenesis of a wide spectrum of metabolic diseases. Understanding the underlying mechanisms of mitochondrial dysfunction and developing effective therapeutic strategies targeting mitochondria are crucial for preventing and treating these diseases. While significant progress has been made in this field, further research is needed to develop more effective and targeted therapies. Future research should focus on identifying novel targets for therapeutic intervention, developing more sophisticated methods for assessing mitochondrial function, and conducting clinical trials to evaluate the efficacy of mitochondrial-targeted therapies.
Many thanks to our sponsor Esdebe who helped us prepare this research report.
References
- Anderson, D. G., & Maechler, P. (2014). Metabolic dysfunction in pancreatic β-cells in type 2 diabetes. Current Opinion in Clinical Nutrition & Metabolic Care, 17(4), 318-326.
- Brand, M. D. (2005). Uncoupling to survive? The role of mitochondrial inefficiency in ageing. Experimental Gerontology, 40(10), 813-820.
- Chan, D. C. (2020). Mitochondrial dynamics and organization in mammals. Cell, 181(2), 309-326.
- Choi, A. M. K., Ryter, S. W., & Levine, B. (2013). Autophagy in human disease. New England Journal of Medicine, 368(7), 651-662.
- Dillin, A., Gottlieb, D., Toivonen, J. M., & Taskent, R. O. (2002). Mitochondrial function and aging. Science, 298(5602), 2398-2401.
- Eckert, A., Keil, U., Scherping, I., Hauptmann, S., Müller, W. E., & Leuner, K. (2003). Stabilization of mitochondrial function as a principle for neuroprotective therapy. Journal of Neurochemistry, 87(1), 1-13.
- Forbes, J. M., & Cooper, M. E. (2013). Mechanisms of diabetic kidney disease. Physiological Reviews, 93(1), 137-188.
- Hardie, D. G. (2011). AMP-activated protein kinase: metabolic sensor and potential drug target. Journal of Internal Medicine, 270(1), 1-22.
- Hood, D. A., & Oliveira, A. N. (2022). Mitochondrial biogenesis in skeletal muscle: molecular mechanisms and physiological relevance. American Journal of Physiology-Endocrinology and Metabolism, 323(1), E1-E18.
- Hwang, S. J., & Moore, K. J. (2021). The role of mitophagy in cardiovascular disease. Journal of the American Heart Association, 10(10), e021054.
- Iqbal, S., Rauf, A., Imran, M., Khan, I. A., Faisal, S. M., Zia-Ul-Haq, M., … & Ur-Rehman, S. (2016). Role of free radicals in various diseases: An updated review. Journal of Cellular Biochemistry, 117(6), 1161-1169.
- Kahn, C. R. (1994). Insulin resistance, insulin sensitivity, and insulin unresponsiveness: a necessary perspective. Diabetes, 43(8), 1066-1075.
- Kang, H. M., Hwang, S., Ryu, D., Seo, W., An, H., Kim, K. H., … & Lee, K. Y. (2018). Mitophagy and insulin resistance. Annals of the New York Academy of Sciences, 1411(1), 53-65.
- Koopman, W. J., Distelmaier, F., Visch, H. J., van den Heuvel, L. P., & Smeitink, J. A. (2012). Oxidative stress in mitochondrial disorders. Biochimica et Biophysica Acta (BBA)-Molecular Basis of Disease, 1822(1), 21-30.
- Murphy, M. P. (2008). Targeting lipophilic cations to mitochondria. Biochimica et Biophysica Acta (BBA)-Bioenergetics, 1777(7-8), 1028-1031.
- Nunnari, J., & Suomalainen, A. (2012). Mitochondria: in sickness and in health. Cell, 148(6), 1145-1159.
- Patten, D. A., & Germain, M. (2017). Mitochondrial DNA in neurodegenerative disorders. *Brain Research Bulletin, 135, 165-172.
- Picard, M., McManus, M. J., Gray, J. D., Nasca, C., Moffat, C., Kopinski, P. K., … & McEwen, B. S. (2015). Mitochondrial functions are acutely sensitive to social stress in mice. Journal of Neuroscience, 35(40), 14023-14035.
- Szendroedi, J., Phielix, E., Klingenspor, M., & Roden, M. (2012). The role of mitochondria in insulin resistance and type 2 diabetes. Nature Reviews Endocrinology, 8(2), 92-103.
- Tahrir, F. G., Ashraf, M. A., Rangasamy, S. B., Abu-Taha, M., & Shafiullah. (2022). Mitochondrial dysfunction, genetic mutations, and associated human diseases. Genes, 13(1), 83.
- Wallace, D. C. (2005). Mitochondrial DNA variation and vertebrate evolution. Trends in Genetics, 21(3), 145-152.
- Youle, R. J., & Narendra, D. P. (2011). Mechanisms of mitophagy. Nature Reviews Molecular Cell Biology, 12(1), 9-14.
The discussion on therapeutic strategies targeting mitochondria is fascinating, especially the emerging mtDNA editing with CRISPR/Cas systems. Overcoming the delivery challenges and minimizing off-target effects could revolutionize treatment for metabolic diseases. What are your thoughts on the ethical considerations surrounding germline mtDNA editing?
That’s a great point! The potential of CRISPR/Cas for mtDNA editing is exciting, and the ethical considerations surrounding germline editing are definitely crucial. Ensuring equitable access, especially given the potential costs, is a key factor in responsible development of this cutting-edge technology. How do we balance innovation with fair access?
Editor: MedTechNews.Uk
Thank you to our Sponsor Esdebe
Gene therapy and stem cell transplants for metabolic disorders? Sounds like an expensive fix for what could be a lifestyle problem. Wonder how long before personalized mitochondrial cocktails are the next big wellness trend?
That’s a thought-provoking point! The idea of “personalized mitochondrial cocktails” as a wellness trend is fascinating, considering the increasing focus on personalized medicine. It highlights how complex research findings can sometimes be interpreted, potentially leading to innovative and, perhaps, unexpected approaches to health optimization. What factors might drive that trend?
Editor: MedTechNews.Uk
Thank you to our Sponsor Esdebe