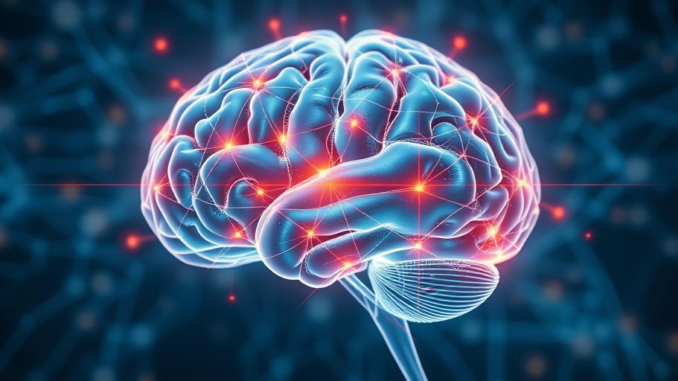
Abstract
Neuroplasticity, the brain’s inherent ability to reorganize its structure, function, and connections in response to intrinsic or extrinsic stimuli, is a cornerstone of neurological development, learning, and recovery from brain injury. This report provides a comprehensive overview of the mechanisms underlying neuroplasticity, including synaptic plasticity, structural plasticity, and neurogenesis. We explore the key modulators influencing these processes, encompassing genetic factors, environmental enrichment, experience-dependent learning, and pharmacological interventions. Furthermore, we critically evaluate the therapeutic potential of harnessing neuroplasticity in various neurological conditions, such as stroke, traumatic brain injury, and neurodegenerative diseases. We discuss both established and emerging strategies, including rehabilitation therapies, brain stimulation techniques, and pharmacological approaches, highlighting the translational challenges and future directions in this rapidly evolving field. Finally, we offer a perspective on the complexities and limitations of neuroplasticity, advocating for a nuanced understanding of its role in health and disease.
Many thanks to our sponsor Esdebe who helped us prepare this research report.
1. Introduction
The concept of the brain as a static and immutable organ has been replaced by the understanding that the brain is a dynamic, adaptable entity capable of continuous remodeling throughout the lifespan. This remarkable capacity, termed neuroplasticity, underlies our ability to learn new skills, adapt to changing environments, and recover from neurological insults. The fundamental basis of neuroplasticity lies in the brain’s intricate network of neurons, synapses, and glial cells, which constantly interact and reorganize in response to various stimuli. The ability of these components to remodel themselves either structurally or functionally allows the brain to adapt to changing circumstances, acquire new abilities, and recover after brain injury or disease.
Understanding the mechanisms and modulators of neuroplasticity is critical for developing effective therapeutic strategies to promote recovery and improve neurological outcomes. Neuroplasticity is not a uniform process; it encompasses a wide range of phenomena, from microscopic changes at the synaptic level to macroscopic alterations in brain structure and function. Furthermore, the effectiveness of neuroplasticity-based interventions is highly dependent on various factors, including the timing and intensity of training, the individual’s genetic background, and the specific neurological condition being treated. This paper aims to provide a comprehensive overview of neuroplasticity, exploring its underlying mechanisms, key modulators, and therapeutic potential, while also highlighting the challenges and limitations in translating basic research findings into clinical practice.
Many thanks to our sponsor Esdebe who helped us prepare this research report.
2. Mechanisms of Neuroplasticity
Neuroplasticity encompasses a diverse array of processes that operate at multiple levels of brain organization. These processes can be broadly categorized into synaptic plasticity, structural plasticity, and neurogenesis.
2.1 Synaptic Plasticity
Synaptic plasticity refers to the activity-dependent changes in the strength and efficacy of synaptic transmission between neurons. This is arguably the most fundamental form of neuroplasticity and is considered the cellular basis of learning and memory. Two prominent forms of synaptic plasticity are long-term potentiation (LTP) and long-term depression (LTD).
LTP involves a persistent strengthening of synaptic connections following high-frequency stimulation. The classical model of LTP involves the activation of NMDA receptors, leading to an influx of calcium ions into the postsynaptic neuron. This calcium influx triggers a cascade of intracellular signaling pathways that result in the insertion of more AMPA receptors into the postsynaptic membrane, thereby increasing the neuron’s sensitivity to subsequent stimulation. LTD, conversely, involves a weakening of synaptic connections following low-frequency stimulation. This weakening is thought to be mediated by a decrease in the number of AMPA receptors in the postsynaptic membrane.
The precise molecular mechanisms underlying LTP and LTD are complex and involve a variety of signaling molecules, including kinases, phosphatases, and transcription factors. Furthermore, the expression of LTP and LTD is highly dependent on the specific brain region, the type of synapse, and the history of synaptic activity. While LTP and LTD are widely studied forms of synaptic plasticity, other mechanisms, such as spike-timing-dependent plasticity (STDP), also play important roles in shaping synaptic connections.
2.2 Structural Plasticity
Structural plasticity refers to the changes in the physical structure of neurons and their connections. This can involve changes in dendritic arborization, spine density, axonal sprouting, and myelination. Structural plasticity is a slower and more complex process than synaptic plasticity, but it can lead to more profound and long-lasting changes in brain organization.
Dendritic arborization, the branching pattern of dendrites, is highly dynamic and can be influenced by experience. Increases in dendritic arborization can increase the surface area available for synaptic connections, thereby enhancing the neuron’s ability to integrate information. Spine density, the number of dendritic spines on a neuron, is also highly plastic and can be modulated by synaptic activity. Spines are the primary sites of excitatory synapses, and changes in spine density can reflect changes in the strength and number of synaptic connections.
Axonal sprouting, the growth of new axonal branches, is another important form of structural plasticity. This process can allow neurons to form new connections with other neurons, thereby rewiring neural circuits. Myelination, the process of wrapping axons with myelin sheaths, can also be modulated by experience. Myelination increases the speed and efficiency of action potential propagation, thereby improving the communication between neurons. Oligodendrocytes are involved in this process and play an important role in the brain’s structural adaptation. Changes in myelin formation or integrity can have profound effects on brain function.
2.3 Neurogenesis
Neurogenesis, the birth of new neurons, was once thought to be limited to early development. However, recent research has demonstrated that neurogenesis continues to occur in specific brain regions throughout adulthood, particularly in the hippocampus and the subventricular zone. New neurons generated in these regions can migrate to other brain areas and integrate into existing neural circuits.
Adult neurogenesis is thought to play a role in learning and memory, particularly in the formation of new memories and the discrimination of similar experiences. It is also implicated in the pathophysiology of various neurological and psychiatric disorders, such as depression and Alzheimer’s disease. The rate of neurogenesis can be influenced by a variety of factors, including exercise, environmental enrichment, and antidepressant medications.
While the contribution of neurogenesis to overall brain plasticity remains a topic of ongoing research, it is clear that the generation of new neurons can contribute to the brain’s ability to adapt and reorganize in response to experience and injury. The interplay between neurogenesis, synaptic plasticity, and structural plasticity is crucial for maintaining brain function and promoting recovery from neurological insults.
Many thanks to our sponsor Esdebe who helped us prepare this research report.
3. Modulators of Neuroplasticity
Neuroplasticity is not a fixed process; its magnitude and direction are influenced by a variety of factors, including genetic predisposition, environmental factors, experience-dependent learning, and pharmacological interventions.
3.1 Genetic Factors
Genetic factors play a significant role in shaping the brain’s capacity for neuroplasticity. Genes involved in synaptic function, neuronal growth, and neurotrophic signaling can influence the individual’s susceptibility to learning, recovery from brain injury, and the development of neurological disorders. Polymorphisms in these genes can lead to variations in brain structure, function, and connectivity, thereby affecting the brain’s ability to adapt to environmental challenges. For example, genes involved in the synthesis and transport of brain-derived neurotrophic factor (BDNF), a key neurotrophin that promotes neuronal survival and plasticity, have been shown to influence learning and memory performance. Similarly, variations in genes involved in synaptic transmission, such as those encoding glutamate receptors, can affect synaptic plasticity and the response to rehabilitation interventions.
3.2 Environmental Enrichment
Environmental enrichment refers to the provision of stimulating and challenging environments that promote exploration, learning, and social interaction. Enriched environments have been shown to enhance neuroplasticity in a variety of ways, including increasing dendritic arborization, spine density, and neurogenesis. These effects are thought to be mediated by increased levels of neurotrophic factors, such as BDNF, and by the activation of intracellular signaling pathways that promote neuronal growth and survival. Environmental enrichment has been shown to improve cognitive function, motor skills, and emotional well-being in both healthy individuals and those with neurological disorders. The effects are not just limited to rodent studies but are mirrored in humans, though the translation of ‘environmental enrichment’ takes on a new form.
3.3 Experience-Dependent Learning
Experience-dependent learning is a powerful driver of neuroplasticity. Repeated exposure to specific stimuli or the performance of specific tasks can lead to changes in brain structure and function that enhance the individual’s ability to perform those tasks more efficiently and effectively. The principles of Hebbian learning, which state that neurons that fire together wire together, provide a theoretical framework for understanding how experience shapes neural circuits. Training-induced neuroplasticity has been demonstrated in a variety of domains, including motor skills, language, and cognitive abilities. The intensity, duration, and specificity of training are critical factors in determining the magnitude and durability of learning-induced neuroplasticity.
3.4 Pharmacological Interventions
Pharmacological interventions can be used to modulate neuroplasticity by targeting specific neurotransmitter systems, signaling pathways, or molecular targets. For example, drugs that enhance cholinergic neurotransmission, such as cholinesterase inhibitors, have been shown to improve cognitive function and enhance synaptic plasticity. Similarly, drugs that modulate glutamate neurotransmission, such as NMDA receptor agonists or antagonists, can influence synaptic plasticity and learning. Other pharmacological agents, such as antidepressants and neurotrophic factors, can promote neuronal survival and growth, thereby enhancing neuroplasticity. The use of pharmacological interventions to enhance neuroplasticity is a promising area of research, but further studies are needed to identify safe and effective drugs and to optimize their use in conjunction with other therapeutic strategies.
3.5 Brain Stimulation Techniques
Brain stimulation techniques are another powerful tool for modulating neuroplasticity. Techniques such as transcranial magnetic stimulation (TMS) and transcranial direct current stimulation (tDCS) can be used to non-invasively stimulate or inhibit specific brain regions, thereby altering neuronal activity and promoting synaptic plasticity. TMS uses magnetic pulses to induce electrical currents in the brain, while tDCS uses weak electrical currents to modulate neuronal excitability. These techniques have been shown to improve motor skills, language abilities, and cognitive function in both healthy individuals and those with neurological disorders. Brain stimulation can be delivered alone or in combination with other therapies, such as rehabilitation exercises, to maximize its effects on neuroplasticity. The mechanisms of action of brain stimulation techniques are complex and involve changes in synaptic transmission, neuronal excitability, and gene expression.
Many thanks to our sponsor Esdebe who helped us prepare this research report.
4. Therapeutic Potential of Neuroplasticity
Harnessing the power of neuroplasticity holds immense therapeutic potential for treating a variety of neurological conditions, including stroke, traumatic brain injury, and neurodegenerative diseases. The goal of neuroplasticity-based therapies is to promote recovery by stimulating the brain to reorganize its structure and function in response to injury or disease.
4.1 Stroke
Stroke is a leading cause of disability, often resulting in motor deficits, language impairments, and cognitive dysfunction. Rehabilitation therapies, such as physical therapy, occupational therapy, and speech therapy, are the cornerstone of stroke recovery. These therapies aim to promote neuroplasticity by providing repetitive and task-specific training that encourages the brain to rewire itself and compensate for damaged areas. Studies have shown that intensive rehabilitation can improve motor function, language abilities, and cognitive function in stroke survivors. The timing of rehabilitation is critical, with early intervention often leading to better outcomes. Furthermore, combining rehabilitation with other therapies, such as brain stimulation or pharmacological interventions, may further enhance neuroplasticity and improve recovery.
4.2 Traumatic Brain Injury
Traumatic brain injury (TBI) can result in a wide range of cognitive, motor, and emotional deficits. The recovery process after TBI is often long and challenging, but neuroplasticity plays a crucial role in helping individuals regain lost function. Rehabilitation therapies, such as cognitive rehabilitation and physical therapy, are essential for promoting neuroplasticity and improving outcomes after TBI. These therapies aim to stimulate the brain to reorganize its structure and function in response to injury. Additionally, pharmacological interventions, such as drugs that promote neuronal survival and growth, may be used to enhance neuroplasticity and improve recovery after TBI. Further research is needed to identify the most effective therapies for promoting neuroplasticity and improving outcomes after TBI.
4.3 Neurodegenerative Diseases
Neurodegenerative diseases, such as Alzheimer’s disease and Parkinson’s disease, are characterized by the progressive loss of neurons and brain function. While these diseases are ultimately progressive and incurable, neuroplasticity can play a role in slowing down the progression of symptoms and improving quality of life. Cognitive training, physical exercise, and social engagement can all stimulate neuroplasticity and help to maintain cognitive and motor function in individuals with neurodegenerative diseases. Additionally, pharmacological interventions, such as drugs that enhance neurotransmitter function or protect neurons from damage, may be used to promote neuroplasticity and slow down the progression of these diseases. Research into novel therapeutic strategies that target specific neuroplasticity mechanisms is ongoing.
Many thanks to our sponsor Esdebe who helped us prepare this research report.
5. Challenges and Future Directions
While the field of neuroplasticity has made significant advances, several challenges remain in translating basic research findings into effective clinical therapies. One of the major challenges is the heterogeneity of neuroplasticity responses. Neuroplasticity is not a uniform process; its magnitude and direction can vary depending on the individual, the specific brain region, the type of injury or disease, and the specific intervention used. Therefore, personalized approaches to neuroplasticity-based therapies are needed to optimize outcomes.
Another challenge is the difficulty in measuring neuroplasticity in vivo. Current techniques for assessing neuroplasticity, such as MRI and EEG, provide only indirect measures of brain structure and function. More sensitive and specific techniques are needed to directly assess synaptic plasticity, structural plasticity, and neurogenesis in living human brains. The advancement of neuroimaging, combined with computational modeling, is essential for future advances.
Future research should focus on identifying the specific molecular mechanisms that underlie neuroplasticity and on developing novel therapeutic strategies that target these mechanisms. This includes exploring the role of non-neuronal cells, such as astrocytes and microglia, in modulating neuroplasticity. Furthermore, more research is needed to optimize the timing, intensity, and specificity of neuroplasticity-based interventions. Combining different therapies, such as rehabilitation, brain stimulation, and pharmacological interventions, may also enhance neuroplasticity and improve outcomes. Finally, more research is needed to understand the long-term effects of neuroplasticity-based interventions and to ensure that these therapies are safe and effective.
Many thanks to our sponsor Esdebe who helped us prepare this research report.
6. Conclusion
Neuroplasticity represents a fundamental property of the brain that allows it to adapt and reorganize in response to experience and injury. Understanding the mechanisms and modulators of neuroplasticity is crucial for developing effective therapeutic strategies to promote recovery and improve neurological outcomes. While significant progress has been made in this field, several challenges remain in translating basic research findings into clinical practice. Future research should focus on identifying the specific molecular mechanisms that underlie neuroplasticity, developing novel therapeutic strategies that target these mechanisms, and optimizing the delivery of neuroplasticity-based interventions. By harnessing the power of neuroplasticity, we can improve the lives of individuals with neurological disorders and enhance the potential for human learning and adaptation.
Many thanks to our sponsor Esdebe who helped us prepare this research report.
References
- Andersen, P., Bliss, T. V., & Skrede, K. K. (1971). Lamellar organization of hippocampal excitatory pathways. Experimental Brain Research, 13(3), 222-238.
- Butefisch, C. (2015). Modulation of cortical plasticity by dopaminergic drugs. Movement Disorders, 30(12), 1577-1589.
- Cramer, S. C. (2008). Repairing the human brain after stroke: I. Mechanisms of spontaneous recovery. Annals of Neurology, 63(3), 272-287.
- Diamond, M. C. (2001). Enriching heredity: The impact of the environment on the anatomy of the brain. Free Press.
- Draganski, B., Gaser, C., Busch, V., Schuierer, G., Bogdahn, U., & May, A. (2004). Neuroplasticity: Changes in grey matter induced by training. Nature, 427(6972), 311-312.
- Fuchs, E., & Flugge, G. (2006). Social stress in rodents: Models and mechanisms. Physiology & Behavior, 89(5), 513-522.
- Gage, F. H. (2002). Neurogenesis in the adult human brain. Nature Reviews Neuroscience, 3(12), 1014-1022.
- Hebb, D. O. (1949). The organization of behavior: A neuropsychological theory. Wiley.
- Kleim, J. A., & Jones, T. A. (2008). Principles of experience-dependent neural plasticity: Implications for rehabilitation after brain injury. Journal of Speech, Language, and Hearing Research, 51(1), S225-S239.
- Lynch, M. A. (2004). Long-term potentiation and memory. Physiological Reviews, 84(1), 87-136.
- Merzenich, M. M., Nelson, R. J., Stryker, M. P., Cynader, M. S., Schoppmann, A., & Zook, J. M. (1984). Somatosensory cortical map changes following digit amputation in adult monkeys. Journal of Comparative Neurology, 224(4), 591-605.
- Nudo, R. J. (2013). Recovery after brain injury: Mechanisms and implications. Frontiers in Human Neuroscience, 7, 887.
- Pascual-Leone, A., Tormos, J. M., Keenan, J., Tarazona, F., Cañete, C., & Catalá, M. D. (1998). Study and modulation of human cortical excitability with transcranial magnetic stimulation. Journal of Clinical Neurophysiology, 15(4), 333-342.
- Rossini, P. M., & Cohen, L. G. (2007). Noninvasive brain stimulation in rehabilitation: An overview of the principles. The Journal of Neuropsychiatry and Clinical Neurosciences, 19(4), 361-370.
- Schmidt-Hieber, C., Jonas, P., & Bischofberger, J. (2004). Action potential initiation and propagation in hippocampal mossy fiber axons. Neuron, 41(5), 727-739.
- Volkmar, N., & Greenough, W. T. (1972). Rearing complexity affects branching of dendrites in the visual cortex of the rat. Science, 176(4033), 1445-1447.
Neurogenesis in adults, huh? So, if I start learning interpretive dance, will I actually grow new brain cells, or just embarrass myself with heightened synaptic connections? Asking for a friend, obviously.
That’s a fantastic question! While the research is still evolving, engaging in complex activities like interpretive dance could certainly stimulate neurogenesis and strengthen existing neural pathways. So, even if your friend experiences a *bit* of embarrassment, they might also be boosting their brainpower! It’s a win-win, really.
Editor: MedTechNews.Uk
Thank you to our Sponsor Esdebe
So, can we just *will* ourselves to be better at parallel parking through sheer neuroplasticity? Asking for a friend who may or may not have driven over a traffic cone yesterday…