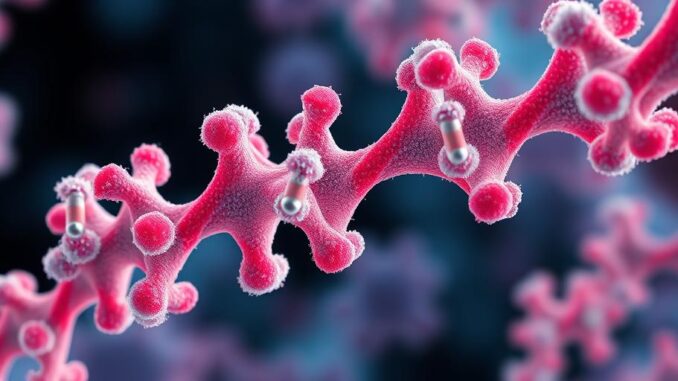
Abstract
Proteins are fundamental macromolecules essential for virtually all biological processes. Their diverse functions stem from their complex three-dimensional structures, which are dictated by their amino acid sequences and influenced by various environmental factors. This report provides a comprehensive overview of protein structure, function, and regulation, exploring the roles of proteins in enzymatic catalysis, cellular signaling, structural support, immune defense, and transport. Furthermore, it delves into the intricate mechanisms governing protein folding, misfolding, and degradation, highlighting the implications of these processes in various diseases, including neurodegenerative disorders, cancers, and metabolic syndromes. The report also examines current and emerging therapeutic strategies targeting proteins, including small molecule inhibitors, antibody-based therapies, and protein engineering approaches, emphasizing the challenges and opportunities associated with these interventions.
Many thanks to our sponsor Esdebe who helped us prepare this research report.
1. Introduction
Proteins are the workhorses of the cell, performing a vast array of functions crucial for life. From catalyzing biochemical reactions to providing structural support and facilitating molecular transport, proteins are indispensable for maintaining cellular homeostasis and orchestrating complex biological processes. Understanding the intricacies of protein structure, function, and regulation is paramount for comprehending the molecular basis of life and developing effective therapeutic interventions for a wide range of diseases. This report aims to provide a comprehensive overview of these aspects, exploring the diverse roles of proteins in biological systems and highlighting the challenges and opportunities associated with targeting proteins for therapeutic purposes.
Many thanks to our sponsor Esdebe who helped us prepare this research report.
2. Protein Structure: Hierarchy and Determinants
Protein structure is organized into four hierarchical levels: primary, secondary, tertiary, and quaternary. The primary structure refers to the linear sequence of amino acids in a polypeptide chain, which is determined by the genetic code. The secondary structure describes the local folding patterns formed by the polypeptide backbone, such as α-helices and β-sheets, stabilized by hydrogen bonds between backbone atoms. The tertiary structure represents the overall three-dimensional arrangement of the polypeptide chain, including the spatial relationships between secondary structure elements and amino acid side chains. This level of structure is stabilized by various non-covalent interactions, including hydrophobic interactions, hydrogen bonds, electrostatic interactions, and van der Waals forces. Some proteins also contain disulfide bonds, covalent linkages between cysteine residues, which contribute to structural stability. Finally, the quaternary structure describes the arrangement of multiple polypeptide chains (subunits) in a multi-subunit protein complex.
The precise folding of a protein into its native conformation is crucial for its function. The amino acid sequence dictates the final three-dimensional structure, but the folding process is also influenced by various environmental factors, including temperature, pH, and the presence of chaperones. Chaperones are proteins that assist in the folding of other proteins, preventing misfolding and aggregation. The hydrophobic effect, which drives the burial of hydrophobic amino acid side chains in the protein core, is a major force in protein folding. However, the complexity of protein folding makes it susceptible to errors, leading to misfolding and aggregation, which can have detrimental consequences for cellular function.
Many thanks to our sponsor Esdebe who helped us prepare this research report.
3. Protein Function: Diverse Roles in Biological Systems
Proteins perform a wide range of functions in biological systems, including:
3.1 Enzymatic Catalysis
Enzymes are proteins that catalyze biochemical reactions, accelerating reaction rates by lowering the activation energy. Enzymes exhibit remarkable specificity, binding to specific substrates and facilitating their conversion into products. The active site of an enzyme is a specific region where the substrate binds and where catalysis occurs. Enzyme activity is regulated by various factors, including substrate concentration, pH, temperature, and the presence of inhibitors or activators. Understanding enzyme mechanisms and regulation is crucial for drug development, as many drugs target specific enzymes involved in disease pathways.
3.2 Cellular Signaling
Proteins play critical roles in cellular signaling pathways, transmitting signals from the cell surface to intracellular targets. Receptor proteins bind to signaling molecules, such as hormones or growth factors, initiating a cascade of intracellular events that ultimately alter gene expression or cellular function. Signaling pathways often involve protein kinases, enzymes that phosphorylate other proteins, thereby modulating their activity. Dysregulation of signaling pathways is implicated in various diseases, including cancer and autoimmune disorders.
3.3 Structural Support
Structural proteins provide support and shape to cells and tissues. Collagen, a fibrous protein, is the major component of connective tissues, providing tensile strength and elasticity. Cytoskeletal proteins, such as actin and tubulin, form filaments that provide structural support to cells and facilitate cell movement and intracellular transport.
3.4 Immune Defense
Antibodies (immunoglobulins) are proteins produced by the immune system that recognize and bind to foreign antigens, such as bacteria and viruses. Antibodies neutralize pathogens, mark them for destruction by immune cells, and activate complement pathways. Other immune proteins, such as cytokines and chemokines, regulate immune cell function and inflammation.
3.5 Transport
Transport proteins facilitate the movement of molecules across cell membranes and throughout the body. Hemoglobin, for example, transports oxygen from the lungs to the tissues. Membrane transport proteins, such as ion channels and transporters, regulate the movement of ions and other molecules across cell membranes.
Many thanks to our sponsor Esdebe who helped us prepare this research report.
4. Protein Misfolding and Aggregation: Implications in Disease
Protein misfolding and aggregation are implicated in a wide range of diseases, including neurodegenerative disorders, such as Alzheimer’s disease, Parkinson’s disease, and Huntington’s disease; prion diseases, such as Creutzfeldt-Jakob disease; and systemic amyloidosis. In these diseases, misfolded proteins accumulate and aggregate, forming toxic oligomers and insoluble fibrils that disrupt cellular function and lead to cell death. The mechanisms underlying protein misfolding and aggregation are complex and involve various factors, including genetic mutations, aging, oxidative stress, and impaired protein degradation pathways.
4.1 Alzheimer’s Disease
In Alzheimer’s disease, the accumulation of amyloid-beta (Aβ) plaques and neurofibrillary tangles composed of hyperphosphorylated tau protein are hallmarks of the disease. Aβ is derived from the amyloid precursor protein (APP) through sequential cleavage by β-secretase and γ-secretase. Misfolded Aβ monomers aggregate to form oligomers, which are believed to be the most toxic species, and eventually form insoluble plaques. Tau protein, a microtubule-associated protein, becomes hyperphosphorylated and misfolds, leading to the formation of neurofibrillary tangles, which disrupt microtubule function and impair neuronal transport.
The precise mechanisms by which Aβ and tau contribute to Alzheimer’s disease pathogenesis are still under investigation. However, evidence suggests that Aβ oligomers trigger a cascade of events, including inflammation, oxidative stress, and synaptic dysfunction, ultimately leading to neuronal cell death. Tau pathology is thought to contribute to the spread of neurodegeneration throughout the brain. Recent research has focused on developing therapies that target Aβ and tau, including antibodies that clear Aβ plaques, inhibitors of β-secretase and γ-secretase, and agents that prevent tau aggregation. The success of these therapies has been limited, likely due to the complexity of the disease and the fact that significant neuronal damage may have already occurred by the time of diagnosis. The recent approval of aducanumab, an anti-Aβ antibody, has been controversial, given the limited evidence of clinical benefit and potential side effects.
4.2 Parkinson’s Disease
Parkinson’s disease is characterized by the loss of dopaminergic neurons in the substantia nigra, a brain region involved in motor control. A hallmark of Parkinson’s disease is the presence of Lewy bodies, intracellular inclusions composed of aggregated α-synuclein protein. α-Synuclein is a neuronal protein whose function is not fully understood, but it is thought to be involved in synaptic vesicle trafficking. Misfolded α-synuclein monomers aggregate to form oligomers and fibrils, which are toxic to neurons. The mechanisms by which α-synuclein aggregation leads to neuronal cell death are not fully understood, but they may involve impaired protein degradation pathways, mitochondrial dysfunction, and oxidative stress.
4.3 Huntington’s Disease
Huntington’s disease is a neurodegenerative disorder caused by an expansion of a CAG repeat in the huntingtin gene, resulting in a protein with an abnormally long polyglutamine tract. The mutant huntingtin protein misfolds and aggregates, forming intracellular inclusions that disrupt neuronal function. The mechanisms by which mutant huntingtin causes neuronal cell death are complex and involve various factors, including impaired protein degradation pathways, mitochondrial dysfunction, and transcriptional dysregulation. Therapies for Huntington’s disease are currently limited to symptomatic treatment.
Many thanks to our sponsor Esdebe who helped us prepare this research report.
5. Protein Degradation Pathways: Quality Control and Cellular Homeostasis
Cells have evolved sophisticated protein degradation pathways to remove misfolded, damaged, or unnecessary proteins, maintaining cellular homeostasis and preventing the accumulation of toxic protein aggregates. The two major protein degradation pathways are the ubiquitin-proteasome system (UPS) and autophagy.
5.1 Ubiquitin-Proteasome System (UPS)
The UPS is the primary pathway for degrading short-lived and misfolded proteins. The process involves tagging proteins with ubiquitin, a small protein, through a series of enzymatic reactions. Polyubiquitinated proteins are then recognized and degraded by the 26S proteasome, a large protein complex that breaks down the protein into small peptides. The UPS plays a critical role in regulating various cellular processes, including cell cycle progression, DNA repair, and immune responses. Dysfunction of the UPS is implicated in various diseases, including cancer and neurodegenerative disorders.
5.2 Autophagy
Autophagy is a catabolic process that involves the engulfment of cytoplasmic components, including organelles and protein aggregates, within double-membrane vesicles called autophagosomes. Autophagosomes then fuse with lysosomes, organelles containing degradative enzymes, where the engulfed material is broken down. Autophagy plays a critical role in removing damaged organelles and protein aggregates, as well as providing nutrients during starvation. There are different types of autophagy, including macroautophagy, microautophagy, and chaperone-mediated autophagy. Dysregulation of autophagy is implicated in various diseases, including cancer, neurodegenerative disorders, and inflammatory diseases.
Many thanks to our sponsor Esdebe who helped us prepare this research report.
6. Therapeutic Strategies Targeting Proteins
Proteins are attractive therapeutic targets due to their central roles in various biological processes. Various therapeutic strategies have been developed to target proteins, including small molecule inhibitors, antibody-based therapies, and protein engineering approaches.
6.1 Small Molecule Inhibitors
Small molecule inhibitors are drugs that bind to specific proteins, inhibiting their activity. Many successful drugs are small molecule inhibitors of enzymes, receptors, or other proteins involved in disease pathways. The development of small molecule inhibitors requires a deep understanding of the protein structure and function, as well as the mechanism of action of the inhibitor. Challenges in small molecule drug development include identifying selective inhibitors that do not have off-target effects, overcoming drug resistance, and ensuring that the drug can reach its target in the body.
6.2 Antibody-Based Therapies
Antibody-based therapies involve the use of antibodies to target specific proteins. Monoclonal antibodies are antibodies that are produced by a single clone of immune cells and recognize a single epitope (a specific region) on a target protein. Antibodies can be used to neutralize proteins, block their interaction with other molecules, or target cells expressing the target protein for destruction by the immune system. Antibody-drug conjugates (ADCs) are antibodies that are linked to cytotoxic drugs, allowing for targeted delivery of the drug to cancer cells. Challenges in antibody-based therapy include developing antibodies that are highly specific and have minimal side effects, overcoming immune responses to the antibody, and ensuring that the antibody can penetrate tissues and reach its target.
6.3 Protein Engineering Approaches
Protein engineering involves modifying the amino acid sequence of a protein to alter its properties, such as its stability, activity, or specificity. Protein engineering can be used to develop novel therapeutic proteins or to improve the properties of existing therapeutic proteins. Techniques used in protein engineering include directed evolution, site-directed mutagenesis, and computational protein design. Challenges in protein engineering include predicting the effects of amino acid mutations on protein structure and function, and ensuring that the engineered protein is stable and functional in vivo.
6.4 Gene Therapy
Although technically not a protein-targeting strategy, gene therapy often aims to modify protein expression. Gene therapy involves introducing genetic material into cells to replace a defective gene or to introduce a new gene that encodes a therapeutic protein. Gene therapy has the potential to treat a wide range of diseases, including genetic disorders, cancer, and infectious diseases. Challenges in gene therapy include delivering the genetic material efficiently and safely to the target cells, ensuring that the gene is expressed at the appropriate level, and preventing immune responses to the gene therapy vector or the expressed protein. Recent advances in CRISPR-Cas9 gene editing technology offer the potential to correct gene defects with greater precision and efficiency.
Many thanks to our sponsor Esdebe who helped us prepare this research report.
7. Challenges and Future Directions
Targeting proteins for therapeutic purposes presents numerous challenges, including the complexity of protein structure and function, the potential for off-target effects, the development of drug resistance, and the difficulty in delivering drugs to the target tissue. Despite these challenges, significant progress has been made in developing protein-based therapies, and there are many opportunities for future innovation. Some key areas for future research include:
- Developing more selective and potent inhibitors: Improving the selectivity and potency of small molecule inhibitors and antibodies can reduce off-target effects and increase efficacy.
- Overcoming drug resistance: Understanding the mechanisms of drug resistance and developing strategies to overcome resistance is crucial for long-term therapeutic success.
- Improving drug delivery: Developing novel drug delivery systems, such as nanoparticles and exosomes, can improve the delivery of drugs to the target tissue and reduce systemic toxicity.
- Developing personalized medicine approaches: Tailoring therapy to the individual patient based on their genetic makeup and disease characteristics can improve treatment outcomes.
- Utilizing computational approaches: Employing computational methods, such as molecular dynamics simulations and machine learning, can accelerate drug discovery and development by predicting protein structure, function, and drug binding.
Furthermore, a deeper understanding of protein-protein interactions (PPIs) is crucial. Disrupting PPIs with small molecules has historically been challenging, but new strategies, such as stapled peptides and PROTACs (PROteolysis TArgeting Chimeras), show promise. PROTACs recruit E3 ubiquitin ligases to target proteins, leading to their degradation via the proteasome. This approach offers a powerful way to reduce the levels of disease-causing proteins, rather than just inhibiting their activity.
The development of more sophisticated protein engineering techniques, such as de novo protein design, could lead to the creation of entirely new therapeutic proteins with tailored properties. This approach involves designing proteins from scratch based on computational models and experimental validation, rather than modifying existing proteins.
Finally, a greater emphasis on early detection and prevention is needed. Identifying biomarkers that can detect protein misfolding and aggregation at early stages of disease could allow for earlier intervention and prevent irreversible neuronal damage. Preventative strategies, such as lifestyle modifications and targeted therapies, could potentially delay or prevent the onset of protein misfolding diseases.
Many thanks to our sponsor Esdebe who helped us prepare this research report.
8. Conclusion
Proteins are essential macromolecules that play diverse roles in biological systems. Understanding their structure, function, and regulation is crucial for comprehending the molecular basis of life and developing effective therapeutic interventions for a wide range of diseases. Protein misfolding and aggregation are implicated in various diseases, and targeting proteins for therapeutic purposes presents numerous challenges. However, significant progress has been made in developing protein-based therapies, and there are many opportunities for future innovation. By focusing on developing more selective and potent inhibitors, overcoming drug resistance, improving drug delivery, utilizing computational approaches, and emphasizing early detection and prevention, we can continue to advance the field of protein-based therapeutics and improve the lives of patients suffering from protein misfolding diseases and other protein-related disorders.
Many thanks to our sponsor Esdebe who helped us prepare this research report.
References
- Anfinsen, C. B. (1973). Principles that govern the folding of protein chains. Science, 181(4096), 223-230.
- Dobson, C. M. (2003). Protein folding and misfolding. Nature, 426(6968), 884-890.
- Gierasch, L. M. (2003). Protein folding: Conformational selection and induced fit. Current Opinion in Structural Biology, 13(5), 562-567.
- Hartl, F. U., Bracher, A., & Hayer-Hartl, M. (2011). Molecular chaperones in protein folding and proteostasis. Nature, 475(7356), 324-332.
- Selkoe, D. J., & Hardy, J. (2016). The amyloid hypothesis of Alzheimer’s disease: progress and problems on the road to therapeutics. Annals of Neurology, 80(5), 621-638.
- De Strooper, B., & Karran, E. (2016). The cellular phase of Alzheimer’s disease. Cell, 164(4), 603-615.
- Goedert, M., Spillantini, M. G., Davies, C. A., Schulz-Schaeffer, W. J., Jakes, R., & Hutton, M. (2000). Filamentous alpha-synuclein inclusions in neurodegenerative diseases. Nature Neuroscience, 3(3), 192-193.
- Rubinsztein, D. C., Mariño, G., & Kroemer, G. (2011). Autophagy and aging. Cell, 146(5), 682-695.
- Savas, J. N., & Jaffrey, S. R. (2021). Proteomics for the study of Alzheimer’s disease. Neuron, 109(14), 2207-2224.
- Poplawski, A., et al. (2019). PROTACs: general strategies for protein degradation. Curr Opin Drug Discov Devel. 22(1):6-17.
- Baker, D. (2020). De novo protein design. Nature, 587(7835), 561-567.
- Paul, S., et al. (2023). Amyloid Plaques and Neurofibrillary Tangles in Alzheimer’s Disease: An Updated Review. International Journal of Molecular Sciences, 24(5), 4314.
So, about these PROTACs targeting proteins for degradation… If we can get proteins to degrade *themselves*, does that mean we’re one step closer to self-cleaning ovens for the body? Asking for a friend… who is also me, and tired of dieting.
That’s a fantastic analogy! The idea of “self-cleaning ovens for the body” is a fun way to think about the potential of targeted protein degradation. While PROTACs don’t quite make proteins degrade *themselves*, they get pretty close by repurposing existing cellular machinery! Perhaps future research can advance these approaches!
Editor: MedTechNews.Uk
Thank you to our Sponsor Esdebe
The discussion of protein misfolding in diseases like Alzheimer’s and Parkinson’s is particularly compelling. Could advancements in chaperone-based therapies or enhanced protein degradation pathways offer more effective methods for managing or preventing these aggregation-related pathologies?