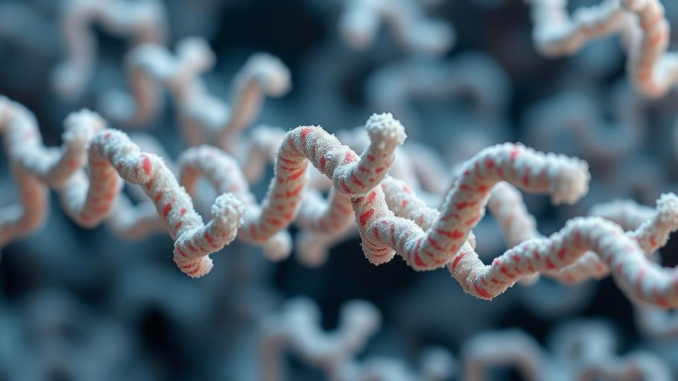
Proteins: Structure, Function, Engineering, and Emerging Applications
Abstract
Proteins are fundamental macromolecules that execute a vast array of biological functions. This review provides an in-depth examination of protein structure, function, engineering strategies, and emerging applications. We explore the hierarchical organization of protein structure from primary amino acid sequence to quaternary assemblies, highlighting the forces driving folding and stability. A comprehensive overview of protein function is presented, encompassing catalysis, transport, signaling, structural support, and immune defense. We delve into the principles of protein engineering, including rational design, directed evolution, and computational approaches, emphasizing their role in tailoring protein properties for specific applications. Finally, we discuss current challenges and future directions in protein research, focusing on areas such as de novo protein design, protein-based therapeutics, and the expanding role of proteins in synthetic biology.
1. Introduction
Proteins, derived from the Greek word proteios meaning “of primary importance,” are the workhorses of the cell, responsible for virtually every aspect of cellular structure, function, and regulation. They are polymers composed of amino acids linked by peptide bonds, forming polypeptide chains that fold into complex three-dimensional structures. The intricate interplay between amino acid sequence and structural conformation dictates the protein’s specific function, allowing for an extraordinary diversity of roles within biological systems. Understanding protein structure, function, and the ability to engineer these molecules is crucial for advancing fields such as medicine, biotechnology, and materials science. This review aims to provide a comprehensive overview of these critical aspects of protein biology, highlighting both established knowledge and emerging frontiers.
2. Protein Structure
The architecture of a protein is typically described at four levels of organization:
-
2.1 Primary Structure: This refers to the linear sequence of amino acids in the polypeptide chain. The primary structure is genetically encoded and determines the protein’s unique identity and subsequent folding pattern. Variations in amino acid sequence can lead to significant alterations in protein function, as exemplified by the single amino acid substitution in hemoglobin that causes sickle cell anemia [1]. Determining the primary structure is crucial for understanding the protein’s evolutionary history, predicting its structural features, and designing synthetic peptides with specific properties.
-
2.2 Secondary Structure: Local regions of the polypeptide chain fold into recurring structural motifs, primarily α-helices and β-sheets. These structures are stabilized by hydrogen bonds between the carbonyl oxygen and amide hydrogen atoms in the peptide backbone. The propensity of an amino acid to form a particular secondary structure element depends on its intrinsic properties and interactions with neighboring residues [2]. Understanding the factors influencing secondary structure formation is essential for predicting protein folding pathways and designing peptides with desired conformational characteristics.
-
2.3 Tertiary Structure: The overall three-dimensional arrangement of all atoms in a single polypeptide chain constitutes the tertiary structure. This structure is stabilized by a variety of non-covalent interactions, including hydrophobic interactions, hydrogen bonds, electrostatic interactions, and van der Waals forces. Disulfide bonds between cysteine residues can also contribute to tertiary structure stability. The tertiary structure determines the protein’s overall shape and is critical for its function. Incorrect folding can lead to aggregation and loss of function, as observed in many neurodegenerative diseases [3].
-
2.4 Quaternary Structure: Some proteins consist of multiple polypeptide chains, or subunits, that assemble to form a functional complex. The arrangement of these subunits in space constitutes the quaternary structure. Subunits can be identical (homo-oligomers) or different (hetero-oligomers). Quaternary structure can influence protein stability, activity, and regulation. For example, hemoglobin is a tetramer consisting of two α-globin and two β-globin subunits, and its oxygen-binding affinity is modulated by cooperative interactions between these subunits [4].
3. Protein Function
The diverse functions of proteins are a direct consequence of their structural complexity and the chemical properties of their amino acid side chains. Key functional categories include:
-
3.1 Catalysis: Enzymes are proteins that catalyze biochemical reactions, accelerating reaction rates by lowering the activation energy. Enzymes exhibit remarkable specificity for their substrates and often employ sophisticated catalytic mechanisms involving active site residues and cofactors [5]. Enzyme kinetics and mechanisms are extensively studied to understand how enzymes function at the molecular level and to design inhibitors for therapeutic purposes.
-
3.2 Transport: Many proteins are involved in transporting molecules across cellular membranes or throughout the body. For example, hemoglobin transports oxygen in the blood, and membrane transporters facilitate the movement of ions and small molecules across cell membranes [6]. The specificity and efficiency of transport proteins are critical for maintaining cellular homeostasis and physiological function.
-
3.3 Signaling: Proteins play key roles in cell signaling pathways, transmitting information from the cell surface to the nucleus and regulating gene expression. Receptor proteins bind to signaling molecules, initiating a cascade of intracellular events that ultimately lead to a cellular response [7]. Dysregulation of signaling pathways can contribute to various diseases, including cancer and autoimmune disorders.
-
3.4 Structural Support: Structural proteins provide support and shape to cells and tissues. For example, collagen is a major component of connective tissue, and keratin forms the structural basis of hair, skin, and nails [8]. The mechanical properties of structural proteins are crucial for maintaining tissue integrity and function.
-
3.5 Immune Defense: Antibodies, also known as immunoglobulins, are proteins that recognize and bind to foreign antigens, such as bacteria and viruses, marking them for destruction by the immune system [9]. Antibodies exhibit remarkable diversity in their antigen-binding specificity, allowing the immune system to recognize and neutralize a wide range of pathogens.
4. Protein Engineering
Protein engineering is the process of designing and modifying proteins to enhance their desirable properties or to create novel functionalities. This can be achieved through a variety of techniques, including:
-
4.1 Rational Design: This approach involves modifying the protein sequence based on a detailed understanding of its structure and function. Site-directed mutagenesis is commonly used to introduce specific amino acid substitutions at targeted positions [10]. Rational design can be used to improve protein stability, enhance catalytic activity, alter substrate specificity, or create new binding sites.
-
4.2 Directed Evolution: This approach mimics the natural process of evolution by subjecting a protein to rounds of mutagenesis and selection for a desired property. Error-prone PCR and DNA shuffling are commonly used to generate libraries of protein variants, which are then screened or selected for improved function [11]. Directed evolution can be used to evolve proteins with enhanced stability, activity, or novel functionalities, even without a detailed understanding of the protein’s structure.
-
4.3 Computational Approaches: Computational methods, such as molecular dynamics simulations and structure prediction algorithms, are increasingly used to aid in protein engineering efforts. These methods can be used to predict the effects of amino acid substitutions on protein structure and function, to design novel protein structures, and to optimize protein-ligand interactions [12]. The accuracy and efficiency of computational methods are constantly improving, making them an increasingly valuable tool for protein engineering.
-
4.4 Semi-Rational Design: This method combines elements of both rational design and directed evolution. It uses computational tools to identify a small set of mutations to test experimentally, then uses directed evolution techniques to find the best variants from the library [13]. This approach reduces the workload associated with each method alone while still producing useful proteins.
5. Emerging Applications of Proteins
Protein engineering and biotechnology have led to a wide range of applications for proteins in various fields:
-
5.1 Protein-Based Therapeutics: Therapeutic proteins, such as antibodies, enzymes, and hormones, are increasingly used to treat a variety of diseases. Antibodies are used in cancer immunotherapy, autoimmune disease therapy, and as antiviral agents [14]. Enzyme replacement therapy is used to treat genetic disorders caused by enzyme deficiencies [15]. Protein-based therapeutics offer several advantages over small molecule drugs, including high specificity, reduced toxicity, and the ability to target complex biological pathways. However, challenges remain in developing stable, bioavailable, and cost-effective protein therapeutics.
-
5.2 Industrial Enzymes: Enzymes are widely used in industrial processes, such as food processing, textile manufacturing, and biofuel production [16]. Engineered enzymes can be used to improve the efficiency, specificity, and sustainability of these processes. For example, cellulases are used to break down cellulose into glucose for biofuel production, and proteases are used in laundry detergents to remove protein stains.
-
5.3 Biosensors: Proteins can be used to create biosensors that detect specific molecules or analytes. Antibodies and enzymes are commonly used as recognition elements in biosensors, providing high sensitivity and specificity [17]. Biosensors are used in a variety of applications, including medical diagnostics, environmental monitoring, and food safety testing.
-
5.4 Protein-Based Materials: Proteins can be used as building blocks for creating novel materials with unique properties. Silk proteins are used to create strong, lightweight fibers, and collagen is used in tissue engineering scaffolds [18]. Engineered proteins can be designed to self-assemble into complex structures with tailored mechanical, optical, and electronic properties.
-
5.5 Synthetic Biology: Proteins are essential components of synthetic biological systems. They are used to build synthetic circuits, create artificial cells, and engineer metabolic pathways [19]. Synthetic biology aims to design and construct biological systems with novel functionalities, and proteins are key players in this endeavor.
6. Challenges and Future Directions
Despite significant advances in protein research, several challenges remain:
-
6.1 Protein Folding Prediction: Predicting the three-dimensional structure of a protein from its amino acid sequence remains a major challenge. While significant progress has been made in recent years with the advent of AlphaFold and other AI-based methods, accurate and reliable structure prediction for all proteins remains an elusive goal [20].
-
6.2 De Novo Protein Design: Designing proteins with novel functions from scratch is a challenging but potentially rewarding area of research. De novo protein design requires a deep understanding of the principles governing protein folding and function, as well as advanced computational tools [21].
-
6.3 Protein Stability and Delivery: Improving the stability and bioavailability of therapeutic proteins remains a significant hurdle. Proteins are often susceptible to degradation by proteases and are poorly absorbed by the body. Strategies for improving protein stability include protein engineering, formulation development, and delivery systems such as liposomes and nanoparticles [22].
-
6.4 Ethical Considerations: The development and use of genetically modified proteins raise ethical concerns related to safety, environmental impact, and accessibility. Careful consideration of these ethical issues is essential to ensure the responsible development and application of protein biotechnology [23].
The future of protein research is bright, with exciting opportunities in areas such as de novo protein design, protein-based therapeutics, and synthetic biology. Advances in computational methods, protein engineering techniques, and materials science will continue to drive innovation in this field, leading to new discoveries and applications that benefit society.
7. Conclusion
Proteins are essential macromolecules with a remarkable diversity of structure and function. Understanding the principles of protein structure, function, and engineering is crucial for advancing fields such as medicine, biotechnology, and materials science. This review has provided an overview of these critical aspects of protein biology, highlighting both established knowledge and emerging frontiers. Continued research and development in this area will undoubtedly lead to new discoveries and applications that address some of the most pressing challenges facing humanity.
References
[1] Ingram, V. M. (1956). A specific chemical difference between the globins of normal human and sickle-cell anaemia haemoglobin. Nature, 178(4525), 792-794.
[2] Chou, P. Y., & Fasman, G. D. (1978). Empirical predictions of protein conformation. Annual review of biochemistry, 47(1), 251-276.
[3] Dobson, C. M. (2003). Protein folding and misfolding. Nature, 426(6968), 884-890.
[4] Perutz, M. F. (1979). Regulation of oxygen affinity of haemoglobin: influence of structure of the globin on the Hb-O2 equilibrium. Annual review of biochemistry, 48(1), 327-386.
[5] Fersht, A. (1999). Structure and mechanism in protein science: a guide to enzyme catalysis and protein folding. Macmillan.
[6] Lodish, H., Berk, A., Zipursky, S. L., Matsudaira, P., Baltimore, D., & Darnell, J. (2000). Molecular cell biology. WH Freeman.
[7] Alberts, B., Johnson, A., Lewis, J., Raff, M., Roberts, K., & Walter, P. (2002). Molecular biology of the cell. Garland science.
[8] Fratzl, P. (2008). Collagen: structure and mechanics. Springer Science & Business Media.
[9] Janeway Jr, C. A., Travers, P., Walport, M., & Shlomchik, M. J. (2001). Immunobiology: the immune system in health and disease. Garland Science.
[10] Wells, J. A. (1990). Additivity of mutational effects in proteins. Biochemistry, 29(37), 8509-8517.
[11] Arnold, F. H. (1998). Directed evolution: bringing new enzymes to life. Accounts of chemical research, 31(3), 125-134.
[12] Baker, D. (2000). Protein structure prediction and design. Nature, 405(6782), 39-42.
[13] Fox, R. J., Davis, S. C., Mundorff, E. C., Newman, L. M., Gavrilovic, V., Goswami, S., & Miller, J. R. (2007). Improving the tolerance of enzymes to solvents. Nature biotechnology, 25(3), 338-344.
[14] Weiner, L. M., Surawski, S. H., & Prewett, M. (2010). Monoclonal antibodies in cancer therapy. Clinical cancer research, 16(6), 1838-1849.
[15] Wraith, J. E. (2006). Lysosomal storage diseases: enzyme replacement therapy. Archives of disease in childhood, 91(1), 7-11.
[16] Kirk, O., Borchert, T. V., & Fuglsang, C. C. (2002). Industrial enzyme applications. Current opinion in biotechnology, 13(4), 345-351.
[17] Turner, A. P. (2013). Biosensors: sense and sensibility. Chemical Society Reviews, 42(8), 3184-3196.
[18] Kaplan, D. L. (2002). Silk as biomaterials. Annual review of materials research, 32(1), 419-461.
[19] Cameron, D. E., Bashor, C. J., & Collins, J. J. (2014). Synthetic biology. Nature Reviews Genetics, 15(1), 1-16.
[20] Senior, A. W., Evans, R., Jumper, J., Kirkpatrick, J., Sifre, L., Green, T., … & Hassabis, D. (2020). Improved protein structure prediction using potentials from deep learning. Nature, 577(7792), 706-710.
[21] Kuhlman, B., Dantas, G., Ireton, G. C., Varani, G., Stoddard, B. L., & Baker, D. (2003). Design of a novel globular protein fold with atomic-level accuracy. Science, 302(5649), 1364-1368.
[22] Duncan, R. (2003). The dawning era of polymer therapeutics. Nature Reviews Drug Discovery, 2(5), 347-360.
[23] Parens, E., & Johnston, J. (2003). Ethical issues in synthetic biology. Woodrow Wilson International Center for Scholars.
De novo protein design, huh? So, we’re basically playing God now, creating life (or at least really complex molecules) from scratch? I wonder if the first protein we design will ironically be an ethics regulator.
That’s a fascinating thought! The idea of an ethics regulator protein is quite meta. It really highlights the potential impact de novo design could have, not just in creating new functions, but also in addressing the ethical implications of the technology itself. It’s definitely something to consider as we move forward in this field.
Editor: MedTechNews.Uk
Thank you to our Sponsor Esdebe