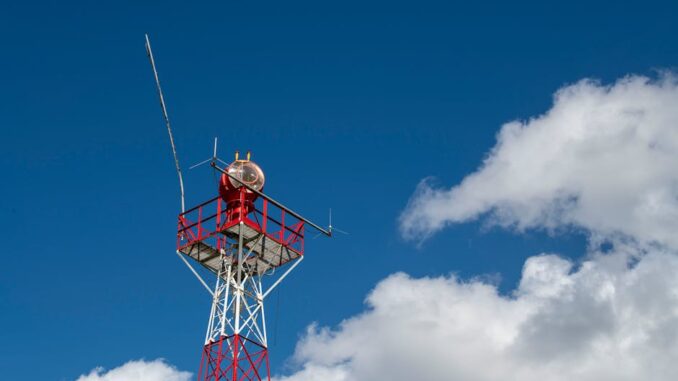
Abstract
Radiation, encompassing both electromagnetic waves and particulate matter, represents a fundamental aspect of the universe. This research report provides a comprehensive overview of radiation, exploring its diverse forms, underlying principles, broad applications across various scientific and technological domains, and the complex biological effects it induces. Beginning with a historical perspective on the discovery and early understanding of radiation, we delve into the electromagnetic spectrum, examining the properties and applications of radio waves, microwaves, infrared, visible light, ultraviolet, X-rays, and gamma rays. We then discuss particle radiation, including alpha, beta, and neutron radiation, detailing their sources, characteristics, and interactions with matter. The report further explores the utilization of radiation in various fields, such as medical imaging and therapy, industrial processes, energy production, and scientific research. A significant portion of the report is dedicated to analyzing the biological effects of radiation, covering both acute and chronic exposures, deterministic and stochastic effects, and the underlying mechanisms of radiation-induced DNA damage, cellular responses, and carcinogenesis. Finally, we discuss current research and future directions in the field of radiation, including advancements in radiation detection and measurement techniques, development of novel radiation sources, strategies for mitigating radiation risks, and the exploration of new applications of radiation in emerging technologies. The report concludes by emphasizing the importance of continued research and responsible utilization of radiation to maximize its benefits while minimizing its potential risks.
Many thanks to our sponsor Esdebe who helped us prepare this research report.
1. Introduction
Radiation, in its broadest sense, is the emission or transmission of energy in the form of waves or particles through space or through a material medium. This phenomenon is ubiquitous, shaping our environment and impacting diverse aspects of our lives. From the light and heat we receive from the sun to the radio waves that enable communication, and the X-rays used in medical imaging, radiation plays a critical role in many fields. Understanding the fundamental principles of radiation, its interactions with matter, and its effects on biological systems is crucial for harnessing its potential benefits while mitigating its associated risks.
The study of radiation has a rich history, dating back to the late 19th century with the discovery of X-rays by Wilhelm Conrad Röntgen in 1895 and radioactivity by Henri Becquerel in 1896. These groundbreaking discoveries revolutionized physics and medicine, leading to the development of new technologies and therapies. However, the early pioneers also faced the harsh realities of radiation exposure, highlighting the importance of understanding the biological effects and implementing safety measures. This report aims to provide a comprehensive overview of radiation, encompassing its various forms, principles, applications, biological effects, and future directions. We will explore both electromagnetic and particulate radiation, examining their sources, properties, and interactions with matter. We will also delve into the applications of radiation in diverse fields, including medicine, industry, energy production, and scientific research. A significant portion of this report will be dedicated to analyzing the biological effects of radiation, covering both acute and chronic exposures, deterministic and stochastic effects, and the underlying mechanisms of radiation-induced damage. Finally, we will discuss current research and future directions in the field of radiation, including advancements in radiation detection and measurement techniques, development of novel radiation sources, strategies for mitigating radiation risks, and the exploration of new applications of radiation in emerging technologies.
Many thanks to our sponsor Esdebe who helped us prepare this research report.
2. Types of Radiation
Radiation can be broadly classified into two main categories: electromagnetic radiation and particle radiation.
2.1 Electromagnetic Radiation
Electromagnetic radiation (EMR) is a form of energy that propagates through space as electromagnetic waves. These waves are characterized by oscillating electric and magnetic fields that are perpendicular to each other and to the direction of propagation. EMR is characterized by its wavelength (λ) and frequency (ν), which are related by the equation: c = λν, where c is the speed of light (approximately 3 x 10^8 m/s). The energy of an electromagnetic wave is quantized and exists in discrete packets called photons. The energy of a photon is given by the equation: E = hν, where h is Planck’s constant (approximately 6.626 x 10^-34 J·s). The electromagnetic spectrum encompasses a wide range of wavelengths and frequencies, each corresponding to a different type of EMR.
- Radio Waves: Radio waves have the longest wavelengths and lowest frequencies in the electromagnetic spectrum. They are used for a wide range of applications, including communication (radio and television broadcasting), radar, and navigation. Different frequencies within the radio wave spectrum are allocated for specific purposes by regulatory bodies.
- Microwaves: Microwaves have shorter wavelengths and higher frequencies than radio waves. They are used in microwave ovens for heating food, in radar systems for detecting objects, and in communication systems for transmitting data.
- Infrared Radiation: Infrared radiation (IR) is associated with heat. It is emitted by objects with a temperature above absolute zero and is used in thermal imaging, remote controls, and industrial heating applications. Different portions of the IR spectrum have different applications, near-IR being used in fiber optics, mid-IR in thermal sensing, and far-IR in spectroscopy.
- Visible Light: Visible light is the portion of the electromagnetic spectrum that is visible to the human eye. It spans a range of wavelengths from approximately 400 nm (violet) to 700 nm (red). Visible light is essential for vision, photosynthesis, and various optical technologies.
- Ultraviolet Radiation: Ultraviolet (UV) radiation has shorter wavelengths and higher frequencies than visible light. It is emitted by the sun and can cause skin damage and other health problems. However, UV radiation is also used in sterilization, medical treatments, and industrial processes. UV is subdivided into UVA, UVB, and UVC, with different biological effects and atmospheric absorption characteristics.
- X-rays: X-rays have much shorter wavelengths and higher frequencies than UV radiation. They are produced when high-energy electrons bombard a target material. X-rays are used in medical imaging to visualize bones and internal organs, in industrial radiography to inspect materials, and in cancer therapy to kill cancer cells.
- Gamma Rays: Gamma rays have the shortest wavelengths and highest frequencies in the electromagnetic spectrum. They are produced by nuclear reactions and radioactive decay. Gamma rays are highly penetrating and can cause significant damage to biological tissues. They are used in cancer therapy to kill cancer cells and in industrial sterilization.
2.2 Particle Radiation
Particle radiation consists of energetic subatomic particles that possess mass and kinetic energy. These particles can interact with matter through various mechanisms, including ionization and excitation.
- Alpha Particles: Alpha particles consist of two protons and two neutrons, which is identical to a helium nucleus. They are emitted during the radioactive decay of heavy nuclei, such as uranium and plutonium. Alpha particles have a high mass and charge, resulting in a short range and high ionization density in matter. They are relatively easily stopped by a sheet of paper or the outer layer of skin.
- Beta Particles: Beta particles are high-energy electrons or positrons emitted during the radioactive decay of certain nuclei. Beta particles have a smaller mass and charge than alpha particles, resulting in a longer range and lower ionization density in matter. They can penetrate several millimeters of tissue but are typically stopped by a thin sheet of aluminum.
- Neutron Radiation: Neutron radiation consists of free neutrons. Neutrons are electrically neutral, which allows them to penetrate deeply into matter. Neutron radiation is produced in nuclear reactors, particle accelerators, and some radioactive decay processes. Neutrons interact with matter through nuclear reactions, which can produce secondary radiation, such as gamma rays.
- Proton Radiation: Protons, positively charged particles found in the nucleus of an atom, can also be accelerated to high energies and used as radiation. Proton therapy is a specialized form of radiation therapy that leverages the Bragg peak effect, where protons deposit the majority of their energy at a specific depth, allowing for targeted treatment of tumors while sparing surrounding healthy tissue.
Many thanks to our sponsor Esdebe who helped us prepare this research report.
3. Sources of Radiation
Radiation sources can be broadly classified into natural and artificial sources.
3.1 Natural Sources of Radiation
Natural sources of radiation are those that exist in the environment without human intervention.
- Cosmic Radiation: Cosmic radiation originates from outer space and consists of high-energy particles, primarily protons and alpha particles. The Earth’s atmosphere and magnetic field provide some protection from cosmic radiation, but the intensity increases with altitude, posing a greater risk to air travelers and astronauts.
- Terrestrial Radiation: Terrestrial radiation comes from radioactive materials present in the Earth’s crust, such as uranium, thorium, and potassium. These materials decay and emit alpha, beta, and gamma radiation. The level of terrestrial radiation varies depending on the geological composition of the area. Radon, a radioactive gas produced by the decay of uranium, is a significant source of terrestrial radiation in indoor environments.
- Internal Radiation: Internal radiation comes from radioactive materials that are ingested or inhaled and become incorporated into the body. These materials can be present in food, water, and air. Naturally occurring radioactive isotopes, such as potassium-40, contribute to internal radiation exposure.
3.2 Artificial Sources of Radiation
Artificial sources of radiation are those that are produced by human activities.
- Medical Applications: Medical applications of radiation include diagnostic imaging (X-rays, CT scans, PET scans) and radiation therapy (external beam radiation, brachytherapy). These procedures expose patients to radiation, but the benefits of diagnosis and treatment generally outweigh the risks.
- Industrial Applications: Industrial applications of radiation include radiography (inspecting welds and materials), gauging (measuring thickness and density), sterilization (medical supplies and food), and irradiation (modifying materials). These applications require careful control of radiation exposure to protect workers and the public.
- Nuclear Power: Nuclear power plants use nuclear fission to generate electricity. The nuclear fuel contains radioactive materials that emit radiation. Nuclear reactors are designed with multiple safety features to prevent the release of radiation into the environment. However, accidents, such as Chernobyl and Fukushima, can result in significant releases of radioactive materials.
- Consumer Products: Some consumer products contain radioactive materials, such as smoke detectors (americium-241) and luminous watches (tritium). These products are designed to be safe under normal use conditions, but improper disposal can pose a risk.
Many thanks to our sponsor Esdebe who helped us prepare this research report.
4. Interactions of Radiation with Matter
The way radiation interacts with matter depends on the type and energy of the radiation, as well as the composition of the material. These interactions lead to the deposition of energy, which can cause various effects, including ionization, excitation, and the breaking of chemical bonds.
4.1 Interactions of Electromagnetic Radiation
- Photoelectric Effect: In the photoelectric effect, a photon interacts with an atom and ejects an electron. The photon is completely absorbed, and the electron gains kinetic energy equal to the photon’s energy minus the binding energy of the electron to the atom. This effect is dominant at low photon energies.
- Compton Scattering: In Compton scattering, a photon interacts with an electron and is scattered at a different angle with reduced energy. The electron gains kinetic energy. This effect is dominant at intermediate photon energies.
- Pair Production: In pair production, a high-energy photon interacts with the electromagnetic field of a nucleus and creates an electron-positron pair. This effect is dominant at high photon energies (above 1.022 MeV).
4.2 Interactions of Particle Radiation
- Ionization: Charged particles, such as alpha and beta particles, interact with matter primarily through ionization. The charged particle passes close to an atom and removes an electron, creating an ion pair (a positive ion and a free electron). The energy required to remove an electron is called the ionization energy.
- Excitation: Charged particles can also excite atoms by transferring energy to an electron, raising it to a higher energy level. The excited atom will then return to its ground state, emitting a photon of light or heat.
- Nuclear Reactions: Neutrons interact with matter primarily through nuclear reactions. A neutron can be absorbed by a nucleus, leading to the formation of a new isotope or the emission of other particles, such as alpha particles or gamma rays. These reactions can produce radioactive isotopes.
Many thanks to our sponsor Esdebe who helped us prepare this research report.
5. Biological Effects of Radiation
Radiation can have various biological effects, depending on the dose, dose rate, type of radiation, and individual sensitivity. These effects can be classified as deterministic or stochastic.
5.1 Deterministic Effects
Deterministic effects are those that have a threshold dose below which no effect is observed. The severity of the effect increases with the dose. Deterministic effects are caused by the death of a large number of cells in a tissue or organ. Examples of deterministic effects include:
- Acute Radiation Syndrome (ARS): ARS occurs after exposure to high doses of radiation over a short period of time. The symptoms of ARS vary depending on the dose and can include nausea, vomiting, fatigue, hair loss, skin burns, and damage to the bone marrow, gastrointestinal tract, and nervous system. ARS can be fatal.
- Cataracts: Cataracts are the clouding of the lens of the eye. Radiation exposure can increase the risk of developing cataracts.
- Sterility: Radiation can damage the reproductive organs and cause sterility.
5.2 Stochastic Effects
Stochastic effects are those that have no threshold dose. Any exposure to radiation carries a risk of developing the effect. The probability of the effect increases with the dose, but the severity of the effect is independent of the dose. Stochastic effects are caused by damage to DNA in individual cells. Examples of stochastic effects include:
- Cancer: Radiation exposure can increase the risk of developing cancer. The risk depends on the dose, dose rate, age at exposure, and type of cancer. Some cancers, such as leukemia and thyroid cancer, are more strongly associated with radiation exposure than others.
- Genetic Effects: Radiation can damage DNA in germ cells (sperm and egg cells), which can lead to genetic mutations that are passed on to future generations. The risk of genetic effects from radiation exposure is generally considered to be low.
5.3 Mechanisms of Radiation Damage
Radiation damages cells primarily by damaging DNA. DNA can be directly damaged by ionization or excitation, or indirectly damaged by free radicals produced by the interaction of radiation with water molecules. This damage can lead to mutations, cell death, or cancer.
- Direct Damage: Direct damage occurs when radiation directly interacts with DNA molecules, causing strand breaks, base damage, or cross-linking. Direct damage is more likely to occur with high-LET radiation, such as alpha particles.
- Indirect Damage: Indirect damage occurs when radiation interacts with water molecules, producing free radicals, such as hydroxyl radicals (OH•). These free radicals are highly reactive and can damage DNA, proteins, and other cellular components. Indirect damage is more likely to occur with low-LET radiation, such as X-rays and gamma rays.
Cells have various mechanisms for repairing DNA damage. However, if the damage is too extensive or if the repair mechanisms are faulty, the cell may undergo apoptosis (programmed cell death) or become cancerous.
Many thanks to our sponsor Esdebe who helped us prepare this research report.
6. Applications of Radiation
Radiation has numerous applications in various fields, including medicine, industry, energy production, and scientific research.
6.1 Medical Applications
- Diagnostic Imaging: X-rays, CT scans, PET scans, and MRI use radiation to create images of the inside of the body. These images are used to diagnose a wide range of medical conditions.
- Radiation Therapy: Radiation therapy uses high-energy radiation to kill cancer cells. Radiation can be delivered externally (external beam radiation) or internally (brachytherapy).
- Sterilization: Radiation is used to sterilize medical supplies and equipment, killing bacteria, viruses, and other microorganisms.
6.2 Industrial Applications
- Radiography: Radiography uses X-rays or gamma rays to inspect materials for defects, such as cracks and voids. It is used in the aerospace, automotive, and construction industries.
- Gauging: Gauging uses radiation to measure the thickness, density, or level of materials. It is used in the paper, plastic, and food industries.
- Sterilization: Radiation is used to sterilize food, killing bacteria, insects, and other pests. It is used to extend the shelf life of food and to prevent foodborne illnesses.
- Irradiation: Irradiation is used to modify the properties of materials, such as plastics and semiconductors. It can be used to increase the strength, durability, or electrical conductivity of materials.
6.3 Energy Production
- Nuclear Power: Nuclear power plants use nuclear fission to generate electricity. Nuclear fission involves splitting the nuclei of heavy atoms, such as uranium, releasing a large amount of energy in the form of heat. This heat is used to boil water, which produces steam that drives turbines to generate electricity.
- Radioisotope Thermoelectric Generators (RTGs): RTGs use the heat generated by the radioactive decay of certain isotopes, such as plutonium-238, to generate electricity. RTGs are used in space probes and remote terrestrial locations where other power sources are not feasible.
6.4 Scientific Research
- Particle Physics: Particle accelerators use radiation to study the fundamental building blocks of matter. These accelerators accelerate particles, such as protons and electrons, to very high energies and collide them with other particles. The resulting collisions produce new particles that can be studied to understand the fundamental forces of nature.
- Materials Science: Radiation is used to study the structure and properties of materials. Techniques such as X-ray diffraction and neutron scattering are used to determine the atomic arrangement and magnetic properties of materials.
- Archaeology: Radiocarbon dating uses the radioactive decay of carbon-14 to determine the age of organic materials. This technique is used to date archaeological artifacts and fossils.
Many thanks to our sponsor Esdebe who helped us prepare this research report.
7. Radiation Detection and Measurement
Accurate detection and measurement of radiation are essential for various applications, including radiation safety, medical diagnostics, and scientific research. Several types of radiation detectors are available, each with its own advantages and limitations.
7.1 Geiger-Müller (GM) Counters
GM counters are widely used for detecting ionizing radiation. They consist of a gas-filled tube with a wire electrode at the center. When ionizing radiation enters the tube, it ionizes the gas, creating a cascade of electrons that produces a pulse of current. GM counters are simple to use and relatively inexpensive, but they cannot distinguish between different types of radiation and have a limited energy resolution.
7.2 Scintillation Detectors
Scintillation detectors use materials that emit light when they interact with ionizing radiation. The amount of light emitted is proportional to the energy deposited by the radiation. The light is detected by a photomultiplier tube (PMT), which converts the light into an electrical signal. Scintillation detectors can be used to detect various types of radiation, including gamma rays, X-rays, and charged particles. They have better energy resolution than GM counters.
7.3 Semiconductor Detectors
Semiconductor detectors use semiconductor materials, such as silicon or germanium, to detect ionizing radiation. When radiation interacts with the semiconductor, it creates electron-hole pairs. These electron-hole pairs are collected by an electric field, producing an electrical signal. Semiconductor detectors have excellent energy resolution and can be used to detect various types of radiation. However, they are more expensive than GM counters and scintillation detectors and often require cooling to operate properly.
7.4 Dosimeters
Dosimeters are used to measure the amount of radiation exposure received by an individual. There are two main types of dosimeters: personal dosimeters and area dosimeters.
- Personal Dosimeters: Personal dosimeters are worn by individuals who work with radiation to monitor their radiation exposure. Common types of personal dosimeters include film badges, thermoluminescent dosimeters (TLDs), and optically stimulated luminescence dosimeters (OSLDS). These dosimeters are processed periodically to determine the cumulative radiation dose received by the individual.
- Area Dosimeters: Area dosimeters are used to monitor the radiation levels in a specific area. They are typically placed in locations where radiation levels may be elevated, such as near radiation sources or in nuclear facilities. Area dosimeters can provide real-time or integrated measurements of radiation levels.
Many thanks to our sponsor Esdebe who helped us prepare this research report.
8. Radiation Safety and Protection
Protecting individuals and the environment from the harmful effects of radiation is of paramount importance. Radiation safety principles are based on the ALARA (As Low As Reasonably Achievable) principle, which aims to minimize radiation exposure while considering practical and economic factors.
8.1 Principles of Radiation Protection
- Time: Minimizing the time spent in the vicinity of a radiation source reduces the radiation exposure. This can be achieved by carefully planning work activities and using remote handling techniques.
- Distance: Increasing the distance from a radiation source significantly reduces the radiation exposure, as radiation intensity decreases with the square of the distance (inverse square law).
- Shielding: Using shielding materials, such as lead, concrete, or water, can effectively attenuate radiation and reduce radiation exposure. The choice of shielding material depends on the type and energy of the radiation.
8.2 Regulatory Framework
Radiation safety is regulated by national and international organizations, such as the International Atomic Energy Agency (IAEA) and national regulatory bodies. These organizations establish radiation safety standards and guidelines, and oversee the licensing and inspection of radiation facilities.
8.3 Emergency Preparedness
Emergency preparedness is essential for responding to radiation accidents and incidents. Emergency plans should include procedures for identifying and assessing the situation, notifying authorities, protecting the public, and mitigating the consequences of the event. Regular drills and exercises should be conducted to ensure that emergency personnel are adequately trained and equipped.
Many thanks to our sponsor Esdebe who helped us prepare this research report.
9. Future Directions
The field of radiation research and technology is constantly evolving, with ongoing efforts to develop new radiation sources, improve radiation detection and measurement techniques, enhance radiation therapy methods, and explore new applications of radiation.
9.1 Advanced Radiation Sources
- Free-Electron Lasers (FELs): FELs are powerful sources of coherent electromagnetic radiation that can be tuned to a wide range of wavelengths, from microwaves to X-rays. FELs have applications in various fields, including materials science, biology, and medicine.
- Laser-Plasma Accelerators (LPAs): LPAs use intense laser pulses to generate plasma waves that can accelerate charged particles to very high energies over short distances. LPAs have the potential to create compact and cost-effective particle accelerators for various applications.
9.2 Improved Radiation Detection and Measurement
- Advanced Scintillation Detectors: Researchers are developing new scintillation materials with improved light output, energy resolution, and radiation resistance. These detectors will enable more sensitive and accurate detection of radiation in various applications.
- Silicon Photomultipliers (SiPMs): SiPMs are solid-state photodetectors that offer several advantages over traditional photomultiplier tubes, including higher sensitivity, lower voltage operation, and immunity to magnetic fields. SiPMs are being used in a wide range of radiation detection applications.
9.3 Enhanced Radiation Therapy
- Proton Therapy: Proton therapy uses protons to deliver radiation to tumors with greater precision than conventional X-ray therapy. Proton therapy can reduce the dose to surrounding healthy tissues and minimize side effects.
- Carbon Ion Therapy: Carbon ion therapy uses carbon ions to deliver radiation to tumors. Carbon ions have a higher linear energy transfer (LET) than protons, which makes them more effective at killing cancer cells that are resistant to conventional radiation therapy.
- FLASH Radiotherapy: FLASH radiotherapy delivers radiation at ultra-high dose rates (hundreds of Gy per second). Studies have shown that FLASH radiotherapy can spare normal tissues while effectively killing cancer cells.
9.4 Emerging Applications
- Quantum Computing: Radiation is being explored as a potential tool for quantum computing. Certain materials, when irradiated, can exhibit quantum properties that could be harnessed for quantum computation.
- Space Exploration: Radiation shielding is a critical aspect of space exploration, especially for long-duration missions. Researchers are developing new radiation shielding materials and strategies to protect astronauts from the harmful effects of cosmic radiation.
Many thanks to our sponsor Esdebe who helped us prepare this research report.
10. Conclusion
Radiation is a fundamental aspect of the universe, with a wide range of applications in various fields. Understanding the principles of radiation, its interactions with matter, and its biological effects is crucial for harnessing its potential benefits while mitigating its associated risks. Continued research and development in radiation science and technology are essential for advancing our knowledge and improving the safety and effectiveness of radiation applications. By adhering to the ALARA principle and implementing appropriate safety measures, we can ensure the responsible utilization of radiation for the benefit of society.
Many thanks to our sponsor Esdebe who helped us prepare this research report.
References
- Attix, F. H. (1986). Introduction to radiological physics and radiation dosimetry. John Wiley & Sons.
- Cember, H., & Johnson, T. E. (2009). Introduction to health physics. McGraw-Hill Professional.
- Hendee, W. R., & Ritenour, E. R. (2002). Medical imaging physics. Wiley-Liss.
- Knoll, G. F. (2010). Radiation detection and measurement. John Wiley & Sons.
- Turner, J. E. (2007). Atoms, radiation, and radiation protection. John Wiley & Sons.
- Hall, E. J., & Giaccia, A. J. (2019). Radiobiology for the Radiologist. Wolters Kluwer.
- IAEA. (2020). International Basic Safety Standards. IAEA Safety Standards Series No. GSR Part 3. Vienna: International Atomic Energy Agency.
- ICRP. (2007). The 2007 Recommendations of the International Commission on Radiological Protection. ICRP Publication 103. Ann. ICRP 37 (2-4).
- United Nations Scientific Committee on the Effects of Atomic Radiation (UNSCEAR). (2008). Sources and effects of ionizing radiation. UNSCEAR 2008 Report to the General Assembly, with scientific annexes. United Nations.
- Wilson, R. L., & Aslam, M. N. (2014). Radioactive isotopes. New York: Momentum Press.
- Durante, M., & Formenti, S. C. (2018). Heavy ions in therapy. Nature Reviews Clinical Oncology, 15(7), 409–423.
- Vozenin, M. C., Hendry, J. H., Limoli, C. L., & Carlson, D. J. (2019). Ultrahigh dose rate (FLASH) radiotherapy: theory and experimental reality. Seminars in Radiation Oncology, 29(1), 3–9.
The report mentions radiocarbon dating for archaeology. Could similar isotopic decay principles be applicable in other fields, perhaps to assess the age and authenticity of non-organic materials like certain polymers or ceramics?