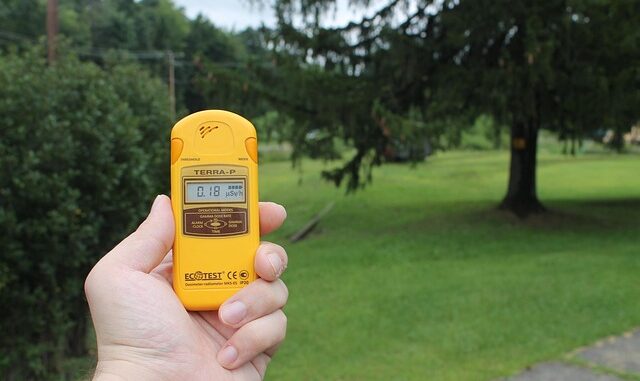
Radiation: A Comprehensive Review of Sources, Biological Effects, Detection, and Mitigation Strategies
Abstract
Radiation, a pervasive phenomenon in the universe, encompasses a broad spectrum of energy propagation, ranging from low-frequency radio waves to high-energy gamma rays and particulate radiation. This review provides a comprehensive overview of radiation, delving into its various sources, the intricate mechanisms governing its interaction with biological systems, the sophisticated methods employed for its detection and quantification, and the diverse strategies developed to mitigate its potentially harmful effects. We explore both ionizing and non-ionizing radiation, emphasizing their distinct characteristics and biological consequences. The report critically assesses the current understanding of radiation-induced DNA damage, cellular stress responses, and long-term health risks, including cancer. Furthermore, we examine the technological advancements in radiation detection, monitoring, and shielding, evaluating their efficacy and limitations in various applications, including medicine, industry, and environmental protection. Finally, we discuss the ethical considerations surrounding radiation exposure and the importance of responsible practices in minimizing risks to human health and the environment.
1. Introduction
Radiation, at its core, represents the emission and propagation of energy through space or a material medium. This energy can manifest as electromagnetic waves (e.g., light, radio waves, X-rays) or as particulate matter (e.g., alpha particles, beta particles, neutrons). The impact of radiation on matter, particularly biological systems, is critically dependent on its energy level and type. Ionizing radiation, characterized by its ability to remove electrons from atoms and molecules, poses a significant threat to living organisms due to its potential to disrupt cellular processes and induce DNA damage. Non-ionizing radiation, while generally less energetic, can still exert biological effects through mechanisms such as thermal heating and the excitation of molecular vibrations.
Understanding radiation is paramount in numerous fields, including medicine (radiotherapy, diagnostic imaging), industry (sterilization, material processing), energy production (nuclear power), and environmental science (radiation monitoring, waste management). The ongoing research into radiation’s fundamental properties, its interactions with matter, and its potential health effects is crucial for developing safer technologies and effective protective measures.
The objective of this review is to provide a detailed and up-to-date analysis of radiation, encompassing its sources, biological effects, detection methods, and mitigation strategies. We aim to offer a comprehensive resource for researchers, policymakers, and practitioners involved in various aspects of radiation science and technology.
2. Sources of Radiation
Radiation sources can be broadly categorized as natural and artificial. Natural sources include cosmic radiation from space, terrestrial radiation from radioactive elements in the Earth’s crust (e.g., uranium, thorium, radon), and internal radiation from radioactive isotopes naturally present in the human body (e.g., potassium-40, carbon-14). Artificial sources, on the other hand, are human-made and include medical X-rays, nuclear power plants, industrial processes, and consumer products (e.g., smoke detectors, some building materials).
2.1 Natural Radiation Sources:
-
Cosmic Radiation: High-energy particles originating from outside the Earth’s atmosphere constantly bombard the planet. The intensity of cosmic radiation varies with altitude and latitude, with higher altitudes and polar regions experiencing greater exposure. The primary particles are protons and alpha particles, but secondary particles, produced through interactions with the atmosphere, also contribute to the radiation dose.
-
Terrestrial Radiation: Radioactive elements like uranium and thorium are naturally present in soil, rocks, and water. These elements decay into other radioactive isotopes, such as radon, which is a colorless, odorless gas that can accumulate in buildings and pose a significant health risk. The concentration of terrestrial radioactive elements varies geographically, with some regions having naturally higher levels than others.
-
Internal Radiation: Radioactive isotopes, such as potassium-40 and carbon-14, are naturally incorporated into the human body through food, water, and air. These isotopes undergo radioactive decay, contributing to the overall internal radiation dose. The magnitude of internal radiation exposure is relatively constant for individuals but can vary slightly based on dietary habits and environmental factors.
2.2 Artificial Radiation Sources:
-
Medical Radiation: Medical imaging techniques, such as X-rays, CT scans, and nuclear medicine procedures, are a significant source of artificial radiation exposure. While these procedures are essential for diagnosis and treatment, it’s crucial to minimize unnecessary radiation exposure by optimizing imaging protocols and using alternative imaging modalities when possible. Advances in imaging technology, such as dose reduction techniques and iterative reconstruction algorithms, have significantly reduced radiation doses in recent years. The use of these technologies must be considered a standard of care to reduce the long-term risk to patient populations.
-
Nuclear Power Plants: Nuclear power plants utilize nuclear fission to generate electricity. During normal operation, these plants release small amounts of radioactive materials into the environment. However, stringent safety regulations and engineering controls are in place to minimize these releases. Accidents at nuclear power plants, such as Chernobyl and Fukushima, can result in significant releases of radioactive materials, leading to widespread environmental contamination and potential health consequences. The legacy of these accidents continues to inform our understanding of radiation risks and the need for robust safety measures.
-
Industrial Radiation: Radiation is used in various industrial applications, including sterilization of medical equipment, food irradiation, non-destructive testing, and gauging. These applications involve the use of radioactive sources or X-ray generators. Strict regulations are in place to ensure the safe handling and disposal of radioactive materials and to minimize the risk of accidental exposure.
3. Biological Effects of Radiation
The biological effects of radiation are complex and depend on factors such as the type of radiation, the dose, the dose rate, the exposed tissue or organ, and the individual’s age and health status. Ionizing radiation can directly damage DNA, proteins, and other cellular components, leading to cell death, mutations, and cancer. Non-ionizing radiation primarily exerts its effects through thermal heating and the excitation of molecular vibrations.
3.1 Mechanisms of Radiation-Induced Damage:
-
Direct Damage: Ionizing radiation can directly interact with DNA molecules, causing strand breaks, base modifications, and cross-linking. Direct damage is particularly significant for high-LET (linear energy transfer) radiation, such as alpha particles and neutrons.
-
Indirect Damage: Ionizing radiation can also interact with water molecules within cells, producing highly reactive free radicals, such as hydroxyl radicals (OH•). These free radicals can then react with DNA, proteins, and other cellular components, causing oxidative damage. Indirect damage is the predominant mechanism for low-LET radiation, such as X-rays and gamma rays.
3.2 Cellular Responses to Radiation:
Cells have evolved various mechanisms to respond to radiation-induced damage, including DNA repair pathways, cell cycle checkpoints, and apoptosis (programmed cell death). The effectiveness of these responses determines the ultimate fate of the cell. If DNA damage is successfully repaired, the cell can survive and continue to function normally. However, if the damage is too extensive or the repair mechanisms are compromised, the cell may undergo apoptosis or become cancerous.
3.3 Acute and Chronic Effects:
-
Acute Effects: High doses of radiation delivered over a short period can cause acute radiation syndrome (ARS), characterized by nausea, vomiting, fatigue, hair loss, and bone marrow suppression. In severe cases, ARS can be fatal. The severity of ARS depends on the radiation dose and the organs affected.
-
Chronic Effects: Long-term exposure to low doses of radiation can increase the risk of cancer, particularly leukemia, thyroid cancer, breast cancer, and lung cancer. The risk of cancer is generally proportional to the cumulative radiation dose. Other chronic effects of radiation exposure include cardiovascular disease, cataracts, and immune system dysfunction. While it is difficult to say with absolute certainty that a cancer is a result of radiation exposure, it is possible to make a statistical assessment of the elevated risk.
3.4 Genetic Effects:
Radiation can cause mutations in germ cells (sperm and eggs), which can be passed on to future generations. These mutations can lead to genetic disorders and developmental abnormalities. While the risk of radiation-induced genetic effects is generally considered to be low, it is a concern, particularly for individuals exposed to high doses of radiation before or during reproductive age.
4. Radiation Detection and Measurement
Accurate detection and measurement of radiation are essential for monitoring radiation levels, assessing radiation exposure, and ensuring the safety of workers and the public. Various types of radiation detectors are available, each with its own advantages and limitations.
4.1 Types of Radiation Detectors:
-
Gas-Filled Detectors: These detectors, such as Geiger-Müller counters and ionization chambers, utilize the ionization of a gas by radiation to produce an electrical signal. Geiger-Müller counters are highly sensitive but cannot distinguish between different types of radiation or measure the energy of the radiation. Ionization chambers are less sensitive but provide a more accurate measure of the radiation dose.
-
Scintillation Detectors: These detectors use materials that emit light when exposed to radiation. The intensity of the light is proportional to the energy of the radiation. Scintillation detectors are widely used in medical imaging and nuclear physics experiments.
-
Semiconductor Detectors: These detectors, such as silicon and germanium detectors, utilize the formation of electron-hole pairs in a semiconductor material by radiation to produce an electrical signal. Semiconductor detectors offer high energy resolution and are used in various applications, including gamma spectroscopy and X-ray analysis.
-
Film Badges and Thermoluminescent Dosimeters (TLDs): These detectors are used for personal radiation monitoring. Film badges contain a photographic film that is exposed to radiation. The degree of darkening of the film is proportional to the radiation dose. TLDs contain materials that store energy when exposed to radiation. The stored energy is released as light when the material is heated. The amount of light released is proportional to the radiation dose.
4.2 Units of Radiation Measurement:
-
Activity: The activity of a radioactive source is the rate at which it decays. The SI unit of activity is the becquerel (Bq), which is defined as one decay per second. The older unit of activity is the curie (Ci), which is equal to 3.7 x 10^10 Bq.
-
Absorbed Dose: The absorbed dose is the amount of energy deposited by radiation in a material per unit mass. The SI unit of absorbed dose is the gray (Gy), which is defined as one joule per kilogram. The older unit of absorbed dose is the rad (radiation absorbed dose), which is equal to 0.01 Gy.
-
Equivalent Dose: The equivalent dose is a measure of the biological effect of radiation, taking into account the type of radiation. It is calculated by multiplying the absorbed dose by a radiation weighting factor (wR). The SI unit of equivalent dose is the sievert (Sv). The older unit of equivalent dose is the rem (roentgen equivalent man), which is equal to 0.01 Sv.
-
Effective Dose: The effective dose is a measure of the overall risk of radiation exposure to the whole body, taking into account the radiosensitivity of different organs and tissues. It is calculated by summing the equivalent doses to each organ or tissue, weighted by a tissue weighting factor (wT). The SI unit of effective dose is also the sievert (Sv).
5. Radiation Shielding and Mitigation Strategies
Protecting individuals and the environment from the harmful effects of radiation requires the implementation of effective shielding and mitigation strategies. These strategies aim to reduce radiation exposure by limiting the source of radiation, increasing the distance from the source, or using shielding materials to absorb or attenuate the radiation.
5.1 Shielding Materials:
-
High-Density Materials: Materials with high densities, such as lead, concrete, and steel, are effective at attenuating gamma rays and X-rays. The effectiveness of a shielding material depends on its density and thickness. Lead is commonly used for shielding in medical and industrial applications due to its high density and relatively low cost. However, the toxicity of lead requires careful handling and disposal. Concrete is a cost-effective shielding material for large-scale applications, such as nuclear power plants and research facilities. Steel is also used for shielding in various applications, particularly where structural strength is required.
-
Neutron Shielding Materials: Neutron shielding requires materials that can effectively slow down and absorb neutrons. Water, polyethylene, and boron-containing materials are commonly used for neutron shielding. Water is an effective neutron moderator, slowing down fast neutrons through collisions with hydrogen atoms. Polyethylene is a lightweight and readily available material that is also effective at neutron moderation. Boron-containing materials, such as borated polyethylene and boron carbide, are effective at absorbing neutrons due to the high neutron capture cross-section of boron.
5.2 Time, Distance, and Shielding:
-
Time: Minimizing the time spent in the vicinity of a radiation source reduces the radiation exposure. This principle is particularly important for workers in radiation-related fields. Careful planning and efficient work practices can significantly reduce radiation exposure.
-
Distance: Increasing the distance from a radiation source reduces the radiation exposure due to the inverse square law. The radiation intensity decreases proportionally to the square of the distance from the source. This principle is applicable in various situations, such as storing radioactive materials at a distance from occupied areas and maintaining a safe distance from X-ray equipment.
-
Shielding: Using shielding materials to absorb or attenuate radiation reduces the radiation exposure. The choice of shielding material depends on the type of radiation, its energy, and the desired level of attenuation. Shielding is an essential component of radiation safety in medical, industrial, and research settings.
5.3 Emergency Response Planning:
Effective emergency response planning is crucial for mitigating the consequences of radiation accidents and incidents. Emergency response plans should include procedures for evacuation, sheltering, decontamination, and medical treatment. Regular drills and training exercises are essential to ensure that emergency responders are prepared to handle radiation emergencies. International organizations, such as the International Atomic Energy Agency (IAEA), provide guidance and support for emergency response planning.
5.4 Decontamination:
Decontamination involves removing radioactive materials from surfaces, equipment, and personnel. Various decontamination techniques are available, including washing, scrubbing, vacuuming, and chemical cleaning. The choice of decontamination technique depends on the type of radioactive contamination and the material being decontaminated. Proper disposal of radioactive waste generated during decontamination is essential to prevent further environmental contamination.
6. Ethical Considerations and Regulatory Framework
The use of radiation technologies raises important ethical considerations, particularly concerning the balance between the benefits of radiation applications and the potential risks to human health and the environment. Responsible practices in radiation safety and the implementation of robust regulatory frameworks are essential to ensure that radiation is used safely and ethically.
6.1 Justification, Optimization, and Limitation:
These three principles are fundamental to radiation protection:
-
Justification: Any activity involving radiation exposure must be justified, meaning that the benefits of the activity must outweigh the risks. This principle requires a careful evaluation of the potential benefits and risks before any decision is made to use radiation.
-
Optimization: Radiation exposures should be kept as low as reasonably achievable (ALARA), taking into account economic and social factors. This principle requires ongoing efforts to reduce radiation exposure by optimizing procedures, using shielding, and minimizing the time spent in the vicinity of radiation sources.
-
Limitation: Radiation doses to individuals must be limited to levels that are considered acceptable by regulatory authorities. Dose limits are based on scientific evidence and are designed to protect individuals from the harmful effects of radiation.
6.2 Regulatory Framework:
Various international and national organizations are responsible for regulating the use of radiation technologies. The IAEA provides international guidance on radiation safety standards and promotes the safe and peaceful use of nuclear energy. National regulatory authorities, such as the Nuclear Regulatory Commission (NRC) in the United States and the Environment Agency in the UK, are responsible for enforcing radiation safety regulations and licensing nuclear facilities. These regulations cover various aspects of radiation safety, including the handling, storage, transportation, and disposal of radioactive materials, as well as the design and operation of radiation-generating equipment.
6.3 Public Communication and Transparency:
Open and transparent communication with the public about radiation risks is essential to building trust and ensuring informed decision-making. Public communication should be based on scientific evidence and should clearly explain the potential benefits and risks of radiation technologies. It is also important to address public concerns and provide opportunities for public input into radiation safety policies.
7. Conclusion
Radiation is a powerful tool with numerous beneficial applications in medicine, industry, and research. However, it also poses potential risks to human health and the environment. A comprehensive understanding of radiation sources, biological effects, detection methods, and mitigation strategies is essential for ensuring the safe and responsible use of radiation technologies. Ongoing research and development efforts are crucial for improving radiation detection and shielding technologies, developing more effective radiation therapies, and minimizing the environmental impact of radiation-related activities. By adhering to ethical principles, implementing robust regulatory frameworks, and promoting open communication with the public, we can harness the benefits of radiation while minimizing its potential risks.
References
- Hall, E. J., & Giaccia, A. J. (2019). Radiobiology for the radiologist. Wolters Kluwer.
- Hendee, W. R., Ritenour, E. R., & Willis, C. E. (2019). Medical imaging physics. John Wiley & Sons.
- IAEA. (2011). International basic safety standards. IAEA Safety Standards Series No. GSR Part 3. Vienna: International Atomic Energy Agency.
- ICRP. (2007). The 2007 recommendations of the International Commission on Radiological Protection. ICRP Publication 103. Ann. ICRP 37 (2-4).
- UNSCEAR. (2008). Effects of ionizing radiation. United Nations Scientific Committee on the Effects of Atomic Radiation. New York: United Nations.
- Bushberg, J. T., Seibert, J. A., Leidholdt Jr, E. M., & Boone, J. M. (2021). The essential physics of medical imaging. Wolters Kluwer.
Given the varying geographical concentrations of terrestrial radiation, are there standardized, publicly accessible maps or databases detailing these levels to inform building material selection and construction practices, particularly regarding radon mitigation?
That’s a great question! Publicly accessible maps detailing terrestrial radiation levels are indeed valuable for informed building material selection. Organizations like the USGS and various geological surveys often provide data on natural background radiation. Sharing this information can significantly contribute to radon mitigation strategies and healthier building practices. This is something we could look into for future research!
Editor: MedTechNews.Uk
Thank you to our Sponsor Esdebe
So, if cosmic radiation increases with altitude, does that mean frequent fliers are practically superheroes in the making? Just wondering how many transatlantic flights I need to develop superpowers…
That’s a fun thought! While transatlantic flights might not turn us into superheroes, you’re right, increased cosmic radiation at altitude is a factor. This increased exposure has implications for flight crew safety, and how frequent fliers are monitored. Perhaps we should look into some research into this!
Editor: MedTechNews.Uk
Thank you to our Sponsor Esdebe
The review’s discussion of medical radiation dose reduction techniques highlights a critical area. Investigating the long-term effects of cumulative low-dose exposure from diagnostic imaging could further refine ALARA principles and inform best practices in radiology.
Thanks for highlighting the importance of dose reduction techniques! You’re right, further research into cumulative low-dose exposure is essential. Refining ALARA principles in radiology based on long-term effects could really optimize diagnostic imaging protocols and minimize patient risk. A deeper dive into this would be invaluable!
Editor: MedTechNews.Uk
Thank you to our Sponsor Esdebe