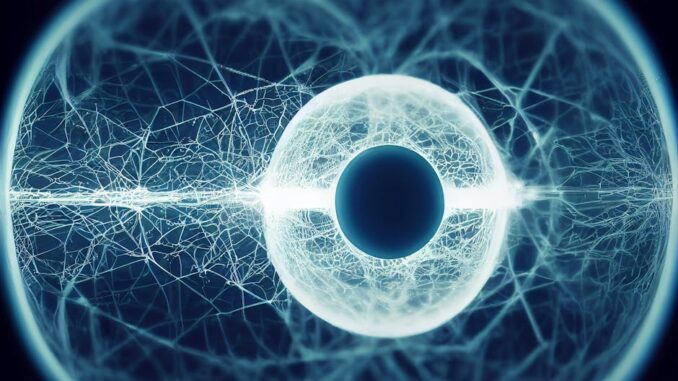
Synaptic Dynamics: From Molecular Mechanisms to Cognitive Resilience and Therapeutic Interventions
Abstract
The synapse, the fundamental unit of communication in the nervous system, is a complex and dynamic structure crucial for all aspects of brain function, from sensory perception to higher-order cognition. This research report provides a comprehensive overview of synaptic biology, exploring its multifaceted nature from molecular mechanisms of neurotransmission to the overarching role in cognitive resilience and disease. We delve into the intricacies of synaptic structure, the dynamics of synaptic plasticity, and the influence of aging on synaptic health. Furthermore, we examine the involvement of synaptic dysfunction in various neurological and psychiatric disorders, with a particular focus on Alzheimer’s disease. Finally, we critically evaluate current and emerging therapeutic strategies aimed at modulating synaptic function, promoting synaptic repair, and mitigating the detrimental effects of synaptic loss. This review highlights the significance of understanding synaptic dynamics for developing effective interventions to maintain cognitive health and combat neurological diseases.
1. Introduction
The human brain, with its estimated 86 billion neurons, relies on trillions of synapses to orchestrate intricate networks that underpin all cognitive processes [1]. These specialized junctions, where neurons communicate with each other, are not static entities but rather highly dynamic structures constantly adapting to experience through a process known as synaptic plasticity [2]. This plasticity, encompassing both structural and functional changes, is the cornerstone of learning, memory, and overall cognitive resilience [3].
However, the delicate balance of synaptic function is vulnerable to disruption from various sources, including aging, genetic predispositions, environmental factors, and disease processes. Synaptic dysfunction is increasingly recognized as a critical early event in the pathogenesis of many neurological disorders, including Alzheimer’s disease (AD), Parkinson’s disease (PD), and Huntington’s disease (HD) [4]. Therefore, understanding the molecular mechanisms governing synaptic function, plasticity, and vulnerability is crucial for developing effective therapeutic strategies aimed at preserving cognitive health and preventing or delaying the onset of neurodegenerative diseases.
This report aims to provide a comprehensive overview of the multifaceted nature of synapses, encompassing their structural organization, functional dynamics, and role in both normal cognitive function and neurological disorders. We will explore the molecular mechanisms underlying synaptic transmission, plasticity, and pruning, as well as the impact of aging on synaptic health. Finally, we will critically evaluate current and emerging therapeutic strategies aimed at enhancing synaptic function and protecting synapses from damage.
2. Synaptic Structure and Molecular Organization
The synapse is a highly specialized structure comprising a presynaptic terminal, a postsynaptic terminal, and the synaptic cleft that separates them. The presynaptic terminal, typically an axon terminal, contains vesicles filled with neurotransmitters, the chemical messengers that transmit signals across the synapse. These vesicles are clustered at specialized release sites called active zones [5]. Upon arrival of an action potential, voltage-gated calcium channels open, triggering an influx of calcium ions that initiates the fusion of synaptic vesicles with the presynaptic membrane, releasing neurotransmitters into the synaptic cleft [6].
The postsynaptic terminal, typically a dendrite or soma, contains receptors that bind to the released neurotransmitters. These receptors can be either ionotropic, directly gating ion channels, or metabotropic, activating intracellular signaling cascades [7]. The postsynaptic density (PSD), a dense protein complex located beneath the postsynaptic membrane, is a critical component of the postsynaptic terminal. The PSD contains a diverse array of scaffolding proteins, signaling molecules, and adhesion molecules that regulate receptor trafficking, synaptic plasticity, and synapse stabilization [8]. Key proteins within the PSD include postsynaptic density protein 95 (PSD-95), which plays a crucial role in anchoring glutamate receptors, and CaMKII, a calcium-dependent kinase involved in synaptic plasticity [9].
The synaptic cleft, a narrow space of approximately 20 nm, is filled with extracellular matrix proteins that play a role in synapse formation and maintenance. Enzymes such as acetylcholinesterase, which degrade neurotransmitters like acetylcholine, are also present in the synaptic cleft to terminate synaptic transmission [10]. The organization of the synapse is highly regulated and dynamic, with continuous trafficking of proteins and lipids between the presynaptic and postsynaptic terminals and the surrounding cell membranes. This dynamic remodeling is essential for synaptic plasticity and adaptation to changing neuronal activity.
3. Synaptic Transmission and Plasticity
Synaptic transmission is the process by which information is transferred from one neuron to another across the synapse. This process involves several key steps, including neurotransmitter synthesis, vesicle packaging, neurotransmitter release, receptor binding, and signal termination [11]. Neurotransmitters, such as glutamate, GABA, dopamine, and serotonin, are synthesized in the presynaptic terminal and packaged into synaptic vesicles by specific vesicular transporters [12]. Upon arrival of an action potential, voltage-gated calcium channels open, triggering calcium influx that activates SNARE proteins (soluble NSF attachment protein receptor). SNARE proteins mediate the fusion of synaptic vesicles with the presynaptic membrane, releasing neurotransmitters into the synaptic cleft [13].
Neurotransmitters diffuse across the synaptic cleft and bind to specific receptors on the postsynaptic membrane. The binding of neurotransmitters to ionotropic receptors directly opens ion channels, leading to rapid changes in membrane potential. The binding of neurotransmitters to metabotropic receptors activates G proteins, which in turn activate intracellular signaling cascades that modulate neuronal excitability and gene expression [14]. The signal is terminated by the removal of neurotransmitters from the synaptic cleft through reuptake by presynaptic transporters or degradation by enzymes. Synaptic plasticity refers to the ability of synapses to strengthen or weaken over time in response to changes in neuronal activity. This plasticity is thought to be the cellular basis of learning and memory [15].
Long-term potentiation (LTP) and long-term depression (LTD) are two well-characterized forms of synaptic plasticity. LTP is a long-lasting increase in synaptic strength, while LTD is a long-lasting decrease in synaptic strength [16]. LTP is typically induced by high-frequency stimulation of the presynaptic neuron, leading to a large influx of calcium ions into the postsynaptic neuron. This calcium influx activates various signaling pathways, including CaMKII and protein kinase C (PKC), which lead to the insertion of more AMPA receptors into the postsynaptic membrane, increasing the postsynaptic response to glutamate [17]. LTD, on the other hand, is typically induced by low-frequency stimulation of the presynaptic neuron, leading to a smaller influx of calcium ions into the postsynaptic neuron. This calcium influx activates different signaling pathways, including protein phosphatases, which lead to the removal of AMPA receptors from the postsynaptic membrane, decreasing the postsynaptic response to glutamate [18].
Synaptic plasticity is not limited to changes in receptor number or function. Structural plasticity, involving changes in the size and shape of dendritic spines and the formation of new synapses, also plays a critical role in learning and memory [19]. The actin cytoskeleton, a dynamic network of protein filaments, is essential for structural plasticity. The activity of various signaling molecules, including Rho GTPases, regulates the polymerization and depolymerization of actin filaments, leading to changes in dendritic spine morphology [20].
4. Synaptic Pruning and Circuit Refinement
Synaptic pruning is a critical developmental process involving the elimination of excess synapses. This process is essential for refining neural circuits and optimizing brain function [21]. During early development, the brain produces an overabundance of synapses. As the brain matures, many of these synapses are eliminated, while the remaining synapses are strengthened and stabilized [22]. Synaptic pruning is guided by neuronal activity and experience. Synapses that are frequently used are strengthened and maintained, while synapses that are rarely used are weakened and eliminated. This process ensures that neural circuits are optimized for the specific demands of the environment [23].
The complement system, a component of the innate immune system, plays a crucial role in synaptic pruning. Complement proteins, such as C1q and C3, tag synapses for elimination by microglia, the resident immune cells of the brain [24]. Microglia engulf and remove the tagged synapses, effectively pruning them from the neural circuit. Dysregulation of synaptic pruning has been implicated in several neurodevelopmental disorders, including autism spectrum disorder (ASD) and schizophrenia [25]. In ASD, excessive synaptic pruning may lead to a reduction in neuronal connectivity, while in schizophrenia, insufficient synaptic pruning may lead to an overabundance of synapses and aberrant neuronal activity. Proper synaptic pruning is essential for the formation of healthy neural circuits and normal brain function.
5. Aging and Synaptic Health
Aging is associated with a progressive decline in synaptic function and a loss of synapses, particularly in brain regions involved in learning and memory, such as the hippocampus and prefrontal cortex [26]. This synaptic loss is thought to contribute to the age-related decline in cognitive function [27]. Several factors contribute to the age-related decline in synaptic health, including oxidative stress, inflammation, mitochondrial dysfunction, and reduced neurotrophic support [28]. Oxidative stress, caused by an imbalance between the production of reactive oxygen species (ROS) and the ability of the brain to detoxify them, can damage synaptic proteins and lipids, leading to synaptic dysfunction [29].
Inflammation, characterized by the activation of microglia and the release of inflammatory cytokines, can also contribute to synaptic dysfunction and loss [30]. Mitochondrial dysfunction, leading to reduced energy production and increased ROS production, can impair synaptic function and increase neuronal vulnerability [31]. Reduced neurotrophic support, such as decreased levels of brain-derived neurotrophic factor (BDNF), can impair synaptic plasticity and survival [32]. Strategies to promote healthy aging of synapses include lifestyle interventions such as exercise, a healthy diet, and cognitive stimulation. These interventions can help to reduce oxidative stress, inflammation, and mitochondrial dysfunction, and increase neurotrophic support, thereby preserving synaptic function and cognitive health [33].
6. Synaptic Dysfunction in Neurological Disorders
Synaptic dysfunction is increasingly recognized as a critical early event in the pathogenesis of many neurological disorders. In Alzheimer’s disease (AD), synaptic loss is strongly correlated with cognitive decline [34]. Amyloid-beta plaques and neurofibrillary tangles, the pathological hallmarks of AD, are thought to disrupt synaptic function and contribute to synaptic loss [35]. Amyloid-beta oligomers can bind to synapses and impair synaptic plasticity, while tau protein, the main component of neurofibrillary tangles, can disrupt axonal transport and impair synaptic function [36]. In Parkinson’s disease (PD), synaptic dysfunction in the basal ganglia contributes to motor deficits [37]. Loss of dopaminergic neurons in the substantia nigra leads to reduced dopamine release in the striatum, disrupting synaptic transmission and impairing motor control. Alpha-synuclein, a protein that aggregates in Lewy bodies in PD, can also impair synaptic function and contribute to synaptic loss [38].
In Huntington’s disease (HD), synaptic dysfunction in the striatum contributes to motor, cognitive, and psychiatric symptoms [39]. The mutant huntingtin protein, which causes HD, can disrupt synaptic transmission and impair synaptic plasticity. Synaptic dysfunction has also been implicated in other neurological disorders, including epilepsy, stroke, and traumatic brain injury [40]. In epilepsy, imbalances in excitatory and inhibitory synaptic transmission can lead to seizures. In stroke, disruption of blood flow to the brain can cause neuronal damage and synaptic loss. In traumatic brain injury, physical trauma to the brain can disrupt synaptic connections and impair synaptic function. Understanding the specific mechanisms of synaptic dysfunction in each of these disorders is crucial for developing effective therapeutic strategies.
7. Therapeutic Strategies Targeting Synapses
Given the critical role of synapses in brain function and neurological disorders, there is growing interest in developing therapeutic strategies that target synapses. These strategies can be broadly divided into three categories: (1) enhancing synaptic function, (2) protecting synapses from damage, and (3) promoting synaptic repair. Strategies to enhance synaptic function include increasing neurotransmitter release, enhancing receptor sensitivity, and promoting synaptic plasticity [41]. Cholinesterase inhibitors, used to treat AD, increase acetylcholine levels in the synaptic cleft by inhibiting the enzyme that breaks down acetylcholine. This can improve cognitive function by enhancing cholinergic neurotransmission [42]. Ampakines, a class of drugs that enhance the activity of AMPA receptors, can improve synaptic plasticity and cognitive function [43]. Strategies to protect synapses from damage include reducing oxidative stress, inflammation, and excitotoxicity [44]. Antioxidants, such as vitamin E and coenzyme Q10, can reduce oxidative stress and protect synapses from damage. Anti-inflammatory drugs, such as nonsteroidal anti-inflammatory drugs (NSAIDs), can reduce inflammation and protect synapses from damage. NMDA receptor antagonists, such as memantine, can reduce excitotoxicity and protect synapses from damage [45].
Strategies to promote synaptic repair include neurotrophic factors, gene therapy, and cell transplantation [46]. Neurotrophic factors, such as BDNF and nerve growth factor (NGF), can promote synaptic growth and survival. Gene therapy can be used to deliver genes encoding neurotrophic factors or other proteins that promote synaptic function. Cell transplantation, such as the transplantation of stem cells, can be used to replace damaged neurons and synapses [47]. In addition to these pharmacological and biological approaches, lifestyle interventions, such as exercise, a healthy diet, and cognitive stimulation, can also promote synaptic health and cognitive function [48]. These interventions can help to reduce oxidative stress, inflammation, and mitochondrial dysfunction, and increase neurotrophic support, thereby preserving synaptic function and cognitive health.
8. Emerging Technologies and Future Directions
Several emerging technologies hold promise for advancing our understanding of synaptic function and developing novel therapeutic strategies. High-resolution imaging techniques, such as super-resolution microscopy and electron microscopy, allow us to visualize synapses at the nanoscale, providing insights into their structure and organization [49]. Optogenetics, a technique that uses light to control neuronal activity, allows us to manipulate synaptic transmission and plasticity with high precision [50]. Genome editing technologies, such as CRISPR-Cas9, allow us to precisely edit genes involved in synaptic function, providing new avenues for therapeutic intervention [51].
Machine learning and artificial intelligence (AI) are also being used to analyze large datasets of synaptic data, identify novel drug targets, and develop personalized treatments [52]. Future research should focus on elucidating the molecular mechanisms underlying synaptic dysfunction in neurological disorders, developing biomarkers for early detection of synaptic loss, and developing novel therapeutic strategies that target synapses. A combination of basic research, translational research, and clinical trials will be necessary to translate these advances into effective treatments for neurological disorders and to promote healthy aging of the brain.
9. Conclusion
Synapses are the fundamental units of communication in the nervous system and play a critical role in all aspects of brain function. Synaptic dysfunction is increasingly recognized as a critical early event in the pathogenesis of many neurological disorders. Understanding the molecular mechanisms governing synaptic function, plasticity, and vulnerability is crucial for developing effective therapeutic strategies aimed at preserving cognitive health and preventing or delaying the onset of neurodegenerative diseases. Current and emerging therapeutic strategies targeting synapses include enhancing synaptic function, protecting synapses from damage, and promoting synaptic repair. Emerging technologies, such as high-resolution imaging, optogenetics, genome editing, and AI, hold promise for advancing our understanding of synaptic function and developing novel therapeutic strategies. Future research should focus on elucidating the molecular mechanisms underlying synaptic dysfunction in neurological disorders, developing biomarkers for early detection of synaptic loss, and developing novel therapeutic strategies that target synapses.
References
[1] Herculano-Houzel, S. (2009). The human brain in numbers: a linearly scaled-up primate brain. Frontiers in Human Neuroscience, 3, 31.
[2] Citri, A., & Malenka, R. C. (2008). Synaptic plasticity: multiple forms, functions, and mechanisms. Neuropsychopharmacology, 33(1), 18-41.
[3] Barco, A., & Spitzer, N. C. (2013). Development and plasticity: convergent molecular evolution? Neuron, 80(2), 323-335.
[4] Selkoe, D. J., & Hardy, J. (2016). The amyloid hypothesis of Alzheimer’s disease: progress and problems on the road to therapeutics. Annals of Neurology, 80(5), 624-638.
[5] Südhof, T. C. (2012). The presynaptic active zone. Neuron, 75(1), 11-25.
[6] Katz, B. (1969). The release of neural transmitter substances. Liverpool University Press.
[7] Nicholls, D. G. (2005). Functions of ion channels in synapses and nerve cells. Advances in Protein Chemistry, 71, 1-30.
[8] Sheng, M., & Hoogenraad, C. C. (2007). The postsynaptic density: a molecular platform for plasticity. Trends in Neurosciences, 30(12), 581-588.
[9] Kennedy, M. B. (2016). Synaptic signaling in learning and memory. Cold Spring Harbor Perspectives in Biology, 8(2), a016785.
[10] Taylor, P. (2003). Anticholinesterase agents. In Goodman & Gilman’s The Pharmacological Basis of Therapeutics (11th ed., pp. 199-216). McGraw-Hill.
[11] Kandel, E. R., Schwartz, J. H., Jessell, T. M., Siegelbaum, S. A., & Hudspeth, A. J. (2012). Principles of Neural Science (5th ed.). McGraw-Hill.
[12] Schuldiner, S., Shirvan, A., & Michael, D. (1995). Vesicular neurotransmitter transporters: from bacteria to humans. Physiological Reviews, 75(2), 369-392.
[13] Jahn, R., & Fasshauer, D. (2012). Molecular machines governing exocytosis of synaptic vesicles. Nature, 490(7419), 201-211.
[14] Lüscher, C., & Slesinger, P. A. (2010). Emerging roles for G protein-gated inwardly rectifying potassium (GIRK) channels in health and disease. Nature Neuroscience, 13(3), 301-310.
[15] Bliss, T. V., & Collingridge, G. L. (1993). A synaptic model of memory: long-term potentiation in the hippocampus. Nature, 361(6407), 31-39.
[16] Malenka, R. C., & Bear, M. F. (2004). LTP and LTD: an embarrassment of riches. Neuron, 44(1), 5-21.
[17] Lisman, J. E., Schulman, H., Cline, H., & Bredt, D. S. (2002). AMPA receptor trafficking and synaptic plasticity. Nature Reviews Neuroscience, 3(8), 573-583.
[18] Bear, M. F. (2003). Bidirectional synaptic plasticity: from theory to reality. Nature Neuroscience, 6(10), 1015-1023.
[19] Yuste, R., & Bonhoeffer, T. (2001). Morphological changes in dendritic spines associated with long-term potentiation. Annual Review of Neuroscience, 24, 1071-1089.
[20] Hotulainen, P., & Hoogenraad, C. C. (2010). Actin in dendritic spines: connecting dynamics to function. Journal of Cell Biology, 189(4), 619-629.
[21] Huttenlocher, P. R. (1979). Synaptic density in human frontal cortex – developmental changes and effects of aging. Brain Research, 163(2), 195-205.
[22] Rakic, P. (1988). Specification of cerebral cortical areas. Science, 241(4862), 170-176.
[23] Lichtman, J. W., & Colman, H. (2000). Synapse elimination and indelible memory. Neuron, 25(2), 269-278.
[24] Stevens, B., Allen, N. J., Vazquez, L. E., Hall, S. S., Brennan, C. W., Fromer, M., … & Barres, B. A. (2007). The classical complement cascade mediates CNS synapse elimination. Cell, 131(6), 1164-1178.
[25] Sekar, A., Bialas, A. R., de Rivera, H. M., Davis, A., Hammond, T. R., Kamitaki, N., … & McCarroll, S. A. (2016). Schizophrenia risk from complex variation of complement component 4. Nature, 530(7589), 177-183.
[26] Morrison, J. H., & Baxter, M. G. (2012). The ageing cortical synapse: hallmarks and implications. Nature Reviews Neuroscience, 13(4), 240-250.
[27] Burke, S. N., & Barnes, C. A. (2006). Neural plasticity in the ageing brain. Nature Reviews Neuroscience, 7(1), 30-40.
[28] Mattson, M. P., & Arumugam, T. V. (2018). Hallmarks of brain aging: mechanistic insights. Trends in Neurosciences, 41(10), 687-701.
[29] Butterfield, D. A., & Halliwell, B. (2019). Oxidative stress, dysfunctional glucose metabolism and Alzheimer’s disease. Nature Reviews Neuroscience, 20(3), 148-166.
[30] Heneka, M. T., Carson, M. J., Khoury, J. E., Landreth, G. E., Brosseron, F., Feinstein, D. L., … & Kummer, M. P. (2015). Neuroinflammation in Alzheimer’s disease. The Lancet Neurology, 14(4), 388-405.
[31] Swerdlow, R. H., Burns, J. M., & Khan, S. M. (2014). The Alzheimer’s disease mitochondrial cascade hypothesis. Journal of Alzheimer’s Disease, 41(S1), S167-S179.
[32] Chao, M. V. (2003). Neurotrophins and their receptors: a convergence point for many signalling pathways. Nature Reviews Neuroscience, 4(4), 299-309.
[33] Hillman, C. H., Erickson, K. I., & Kramer, A. F. (2008). Be smart, exercise your heart: exercise effects on brain and cognition. Nature Reviews Neuroscience, 9(1), 58-65.
[34] DeKosky, S. T., Scheff, S. W., & Styren, S. D. (1996). Structural correlates of cognition in dementia: implications for therapeutic strategies. Alzheimer Disease & Associated Disorders, 10 Suppl 1, 54-61.
[35] Mucke, L., & Selkoe, D. J. (2012). Neurotoxicity of amyloid β-protein: synaptic and network dysfunction. Neuron, 75(5), 777-791.
[36] Iqbal, K., Liu, F., Gong, C. X., & Grundke-Iqbal, I. (2010). Tau in Alzheimer disease and related tauopathies. Current Alzheimer Research, 7(8), 656-664.
[37] Surmeier, D. J., Graves, S. M., Eberhard, D., & Shumilla, J. (2017). Parkinson’s disease and the basal ganglia: a systems perspective. Current Opinion in Neurobiology, 44, 135-142.
[38] Lashuel, H. A., Petrucelli, L., & Kopito, R. R. (2013). α-Synuclein, genetics, and Parkinson’s disease: expanding the pathogenic landscape. Nature Reviews Neuroscience, 14(6), 381-400.
[39] Raymond, L. A., Andre, V. M., Cepeda, C., & Levine, M. S. (2011). Pathophysiology of Huntington’s disease. Trends in Neurosciences, 34(2), 85-93.
[40] Lozano, A. M., Lipsman, N., Bergman, H., Eisner, A., Hutchison, W. D., & Dostrovsky, J. O. (2008). Parkinson’s disease: converging on the subthalamic nucleus. The Lancet Neurology, 7(3), 209-217.
[41] Lynch, M. A. (2004). Long-term potentiation and memory. Physiological Reviews, 84(1), 87-136.
[42] Birks, J. (2006). Cholinesterase inhibitors for Alzheimer’s disease. Cochrane Database of Systematic Reviews, (1), CD005593.
[43] Lynch, G. (2002). Long-term potentiation and its impact on theories of learning and memory. Brain Research Bulletin, 57(3-4), 611-618.
[44] Butterfield, D. A., Poon, H. F., & Sultana, R. (2006). Redox proteomics in brain aging: understanding the effects of oxidative stress on the progression of Alzheimer’s disease. Journal of Alzheimer’s Disease, 10(1), 5-15.
[45] Lipton, S. A. (1999). Ischemic cell death: an NMDA receptor-mediated paradigm. Stroke, 30(8 Suppl), I72-I78.
[46] Varon, S., & Adler, R. (1980). Nerve growth factor and control of nerve cell development. Critical Reviews in Biochemistry, 8(3), 221-244.
[47] Lindvall, O., & Hagell, P. (2000). Cell therapy for Parkinson’s disease. Nature Reviews Neuroscience, 1(1), 65-73.
[48] Cotman, C. W., & Berchtold, N. C. (2002). Exercise: a behavioral intervention to enhance brain health and plasticity. Trends in Neurosciences, 25(6), 295-301.
[49] Hell, S. W. (2007). Far-field optical nanoscopy. Science, 316(5828), 1153-1158.
[50] Deisseroth, K. (2015). Optogenetics: 10 years of microbial opsins in neuroscience. Nature Neuroscience, 18(9), 1213-1225.
[51] Doudna, J. A., & Charpentier, E. (2014). Genome editing. The new frontier of genome engineering with CRISPR-Cas9. Science, 346(6213), 1258096.
[52] Esteva, A., Kuprel, B., Novoa, R. A., Ko, J., Swani, S. M., Blau, H. M., … & Threlfall, C. J. (2017). Dermatologist-level classification of skin cancer with deep neural networks. Nature, 542(7639), 115-118.
This is a great overview of synaptic dynamics. Considering the role of synaptic pruning in refining neural circuits, how might early childhood interventions impact synaptic development and subsequent cognitive abilities later in life?
Thanks for your insightful comment! It’s fascinating to consider how early interventions can shape synaptic development. Enriched environments or targeted therapies during critical periods might enhance beneficial pruning, leading to stronger, more efficient neural circuits and improved cognitive outcomes later on. Further research is needed in this area!
Editor: MedTechNews.Uk
Thank you to our Sponsor Esdebe
Fascinating deep dive into synapses! Makes you wonder, if we could 3D print synapses, could we then upgrade our brains like we upgrade our phones? Asking for a friend, of course.
That’s a thought-provoking question! The idea of “upgrading” our brains is certainly science fiction territory now, but it highlights how much we’re learning about the intricate relationship between synaptic structure and function. If we could replicate the complex molecular environment of a synapse, who knows what cognitive enhancements might be possible one day?
Editor: MedTechNews.Uk
Thank you to our Sponsor Esdebe