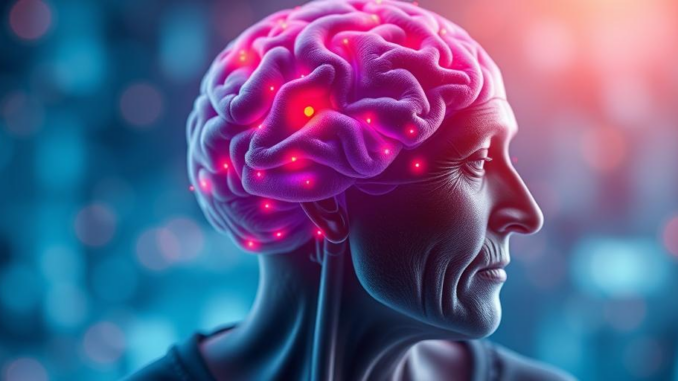
Abstract
Alzheimer’s disease (AD) is a devastating neurodegenerative disorder characterized by progressive cognitive decline. While amyloid plaques and neurofibrillary tangles have long been considered hallmarks of the disease, accumulating evidence points to synaptic dysfunction and loss as early and critical drivers of cognitive impairment. This report delves into the concept of synaptic resilience – the ability of synapses to maintain function despite pathological insults – as a crucial factor in determining AD pathogenesis and a promising target for therapeutic intervention. We explore the molecular and cellular mechanisms underlying synaptic resilience, examining the roles of various signaling pathways, structural proteins, and homeostatic processes. Furthermore, we discuss the influence of genetic and environmental factors on synaptic vulnerability and resilience. Finally, we review emerging therapeutic strategies aimed at bolstering synaptic function and protecting against synaptic loss in AD, considering both pharmacological and non-pharmacological approaches. A greater understanding of synaptic resilience offers hope for developing novel interventions that can delay disease progression and improve the quality of life for individuals affected by AD.
Many thanks to our sponsor Esdebe who helped us prepare this research report.
1. Introduction
Alzheimer’s disease (AD), the most prevalent form of dementia, poses a significant global health challenge. The disease is characterized by a gradual decline in cognitive functions, including memory, language, and executive abilities, ultimately leading to severe disability and dependence. The neuropathological hallmarks of AD include the accumulation of amyloid-beta (Aβ) plaques and neurofibrillary tangles (NFTs) composed of hyperphosphorylated tau protein in the brain. These pathological features were initially considered the primary drivers of neurodegeneration and cognitive decline. However, increasing evidence suggests that synaptic dysfunction and loss occur early in the disease process, even before the formation of widespread plaques and tangles, and are more closely correlated with cognitive impairment than either Aβ or tau pathology alone [1, 2].
The “amyloid cascade hypothesis” has long dominated AD research, positing that Aβ accumulation triggers a cascade of events leading to tau hyperphosphorylation, neuroinflammation, and ultimately, neuronal loss. However, clinical trials targeting Aβ have yielded limited success, prompting a reevaluation of the underlying mechanisms of AD pathogenesis and a shift towards earlier interventions that address synaptic dysfunction [3]. Synapses, the junctions between neurons that allow for communication, are highly dynamic structures capable of adapting to changing environmental demands through a process known as synaptic plasticity. This plasticity is essential for learning, memory, and other cognitive functions. However, in AD, synaptic plasticity is impaired, and synapses become vulnerable to damage.
This report focuses on the concept of synaptic resilience, which refers to the ability of synapses to maintain their function and structure despite exposure to pathological insults, such as Aβ, tau, and inflammation. Synaptic resilience is not a static property but rather a dynamic state influenced by a complex interplay of genetic, epigenetic, and environmental factors. Enhancing synaptic resilience is emerging as a promising therapeutic strategy for preventing or slowing down the progression of AD. This report will delve into the molecular and cellular mechanisms underlying synaptic resilience, explore the factors that modulate it, and discuss potential therapeutic approaches for promoting synaptic health in the context of AD.
Many thanks to our sponsor Esdebe who helped us prepare this research report.
2. Mechanisms of Synaptic Dysfunction in Alzheimer’s Disease
Synaptic dysfunction in AD is a multifaceted process driven by several interacting factors. Aβ oligomers, soluble aggregates of Aβ, are considered to be potent synaptotoxins. They can disrupt synaptic transmission, impair synaptic plasticity, and trigger synaptic loss through various mechanisms, including:
- Impaired receptor trafficking: Aβ oligomers can interfere with the trafficking of glutamate receptors, such as AMPA receptors and NMDA receptors, to the synapse, leading to reduced synaptic transmission and impaired long-term potentiation (LTP), a cellular model of learning and memory [4].
- Disruption of synaptic structure: Aβ oligomers can disrupt the structural integrity of synapses by interacting with synaptic proteins, such as synaptophysin and PSD-95, leading to synaptic disassembly and loss [5].
- Activation of inflammatory pathways: Aβ oligomers can activate microglia and astrocytes, leading to the release of pro-inflammatory cytokines and chemokines that contribute to synaptic dysfunction and neurodegeneration [6].
Tau, a microtubule-associated protein, also plays a crucial role in synaptic dysfunction. In AD, tau becomes hyperphosphorylated and misfolded, forming NFTs. However, soluble tau oligomers and phosphorylated tau can also impair synaptic function by:
- Disrupting axonal transport: Hyperphosphorylated tau can disrupt axonal transport, leading to the accumulation of proteins and organelles in the cell body and a reduction in the delivery of essential molecules to the synapse [7].
- Impairing synaptic plasticity: Tau pathology can impair LTP and long-term depression (LTD), cellular models of learning and memory, by interfering with the signaling pathways involved in synaptic plasticity [8].
- Activating inflammatory pathways: Similar to Aβ, tau pathology can activate microglia and astrocytes, leading to the release of pro-inflammatory mediators that contribute to synaptic dysfunction [9].
In addition to Aβ and tau, other factors contribute to synaptic dysfunction in AD, including:
- Mitochondrial dysfunction: Impaired mitochondrial function can lead to reduced energy production, increased oxidative stress, and impaired calcium homeostasis, all of which can disrupt synaptic transmission and plasticity [10].
- Impaired calcium homeostasis: Dysregulation of calcium homeostasis can lead to excitotoxicity, a process in which excessive stimulation of glutamate receptors leads to neuronal damage and death [11].
- Neuroinflammation: Chronic neuroinflammation can exacerbate synaptic dysfunction and neurodegeneration by releasing pro-inflammatory mediators and reactive oxygen species [12].
Understanding the complex interplay of these factors is crucial for developing effective therapeutic strategies that target synaptic dysfunction in AD.
Many thanks to our sponsor Esdebe who helped us prepare this research report.
3. Molecular and Cellular Mechanisms of Synaptic Resilience
Synaptic resilience is the ability of synapses to withstand pathological insults and maintain their function and structure. This resilience is not a passive property but rather an active process involving a complex interplay of molecular and cellular mechanisms. Several key factors contribute to synaptic resilience:
- Enhanced synaptic plasticity: Synapses with a high capacity for plasticity are better able to adapt to changing conditions and compensate for damage. This enhanced plasticity can be achieved through various mechanisms, including increased expression of glutamate receptors, enhanced LTP, and reduced LTD [13].
- Increased expression of neurotrophic factors: Neurotrophic factors, such as brain-derived neurotrophic factor (BDNF) and nerve growth factor (NGF), promote neuronal survival, growth, and differentiation and enhance synaptic function. Increased expression of these factors can protect synapses from damage and promote their repair [14].
- Efficient clearance of Aβ and tau: Efficient clearance of Aβ and tau from the brain can reduce the exposure of synapses to these toxic proteins and prevent synaptic dysfunction. This clearance can be achieved through various mechanisms, including enhanced activity of Aβ and tau degrading enzymes and improved function of the glymphatic system, a brain-wide waste clearance system [15].
- Improved mitochondrial function: Healthy mitochondria are essential for providing the energy needed to maintain synaptic function. Improving mitochondrial function can protect synapses from damage and enhance their resilience [16].
- Enhanced antioxidant defense: Oxidative stress can damage synapses and contribute to synaptic dysfunction. Enhanced antioxidant defense mechanisms can protect synapses from oxidative damage and improve their resilience [17].
-
Regulation of Calcium Homeostasis: Precise regulation of calcium signaling is crucial for synaptic function and plasticity. Mechanisms that maintain proper calcium levels within synapses, such as calcium buffering proteins and efficient calcium extrusion pathways, contribute to synaptic resilience [18]. Disruptions in calcium homeostasis are strongly linked to AD pathogenesis, and maintaining proper calcium regulation can enhance synaptic resilience against pathological insults.
-
Structural Synaptic Proteins: The cytoskeleton and scaffolding proteins, like actin, tubulin, and PSD-95, provide structural support to synapses. Upregulation and stabilization of these proteins reinforce synaptic connections, increasing their resistance to disruption by Aβ or tau. Furthermore, proteins involved in synapse formation and maintenance, such as neuroligins and neurexins, play a key role in maintaining synaptic integrity under pathological conditions [19].
-
Homeostatic Synaptic Plasticity: This type of plasticity ensures that overall neuronal activity remains within a physiological range. It involves scaling synaptic strength up or down to compensate for changes in network activity. In the context of AD, homeostatic mechanisms can help maintain neuronal firing rates and prevent over-excitation or silencing of neurons, contributing to synaptic resilience by mitigating the effects of aberrant activity [20].
Understanding these mechanisms is crucial for developing therapeutic strategies that enhance synaptic resilience and protect against synaptic loss in AD.
Many thanks to our sponsor Esdebe who helped us prepare this research report.
4. Genetic and Environmental Modulators of Synaptic Resilience
Synaptic resilience is influenced by a complex interplay of genetic and environmental factors. Genetic factors can affect the expression and function of proteins involved in synaptic plasticity, neurotrophic signaling, Aβ and tau clearance, mitochondrial function, and antioxidant defense. For example:
- APOE genotype: The apolipoprotein E (APOE) gene has three common alleles: APOE2, APOE3, and APOE4. The APOE4 allele is the strongest genetic risk factor for late-onset AD, while the APOE2 allele is protective. APOE4 has been shown to impair Aβ clearance, promote tau pathology, and disrupt synaptic function, leading to increased synaptic vulnerability. In contrast, APOE2 may enhance Aβ clearance and protect synapses from damage [21].
- TREM2 variants: Triggering receptor expressed on myeloid cells 2 (TREM2) is a receptor expressed on microglia that plays a role in immune response and Aβ clearance. Rare variants in TREM2 have been associated with an increased risk of AD. These variants can impair microglial function, leading to reduced Aβ clearance and increased neuroinflammation, which can contribute to synaptic dysfunction [22].
- Other genetic variants: Several other genetic variants have been associated with AD risk, including variants in genes involved in endosomal trafficking, lipid metabolism, and immune function. These variants may also affect synaptic resilience by influencing various cellular processes [23].
Environmental factors can also significantly impact synaptic resilience. Lifestyle factors, such as diet, exercise, and cognitive activity, can influence synaptic function and vulnerability. For example:
- Diet: A healthy diet, rich in fruits, vegetables, and omega-3 fatty acids, has been associated with a reduced risk of AD. These dietary components may enhance synaptic function by promoting neurotrophic signaling, reducing inflammation, and protecting against oxidative stress. Conversely, a diet high in saturated fat and sugar has been linked to an increased risk of AD [24].
- Exercise: Regular physical exercise has been shown to improve cognitive function and reduce the risk of AD. Exercise can enhance synaptic plasticity by increasing the expression of BDNF and other neurotrophic factors [25].
-
Cognitive activity: Engaging in mentally stimulating activities, such as reading, puzzles, and social interaction, can help maintain cognitive function and reduce the risk of AD. Cognitive activity may enhance synaptic resilience by promoting synaptic plasticity and strengthening neural networks [26].
-
Sleep: Adequate sleep is crucial for synaptic homeostasis and consolidation of memories. Sleep deprivation has been linked to increased Aβ levels and impaired synaptic function. Maintaining a regular sleep schedule and ensuring sufficient sleep duration can support synaptic resilience and reduce the risk of AD [27].
-
Stress: Chronic stress can impair synaptic plasticity and increase vulnerability to neurodegeneration. Managing stress through techniques like mindfulness, meditation, or yoga can help protect synapses and enhance resilience [28].
Understanding the interplay of genetic and environmental factors that influence synaptic resilience is crucial for developing personalized prevention strategies that can reduce the risk of AD.
Many thanks to our sponsor Esdebe who helped us prepare this research report.
5. Therapeutic Strategies Targeting Synaptic Resilience
Given the importance of synaptic dysfunction in AD pathogenesis, therapeutic strategies aimed at enhancing synaptic resilience are gaining increasing attention. These strategies can be broadly classified into pharmacological and non-pharmacological approaches.
5.1 Pharmacological Approaches
- Drugs that enhance neurotrophic signaling: Several drugs are being developed to enhance neurotrophic signaling, such as BDNF and NGF. These drugs can promote neuronal survival, growth, and differentiation and enhance synaptic function. For example, LM11A-31, a small molecule that selectively activates the p75NTR receptor, has shown promise in preclinical studies for protecting synapses from Aβ-induced damage [29].
- Drugs that modulate synaptic plasticity: Drugs that modulate synaptic plasticity, such as AMPAkines (positive allosteric modulators of AMPA receptors), can enhance LTP and improve cognitive function. However, the use of AMPAkines in AD has been limited by their potential for excitotoxicity [30].
- Drugs that target Aβ and tau: While targeting Aβ and tau has not been consistently successful in clinical trials, some agents show promise in reducing the levels of these toxic proteins and protecting synapses from damage. For example, monoclonal antibodies that target Aβ oligomers have shown some efficacy in reducing Aβ plaques and slowing cognitive decline [31]. Tau aggregation inhibitors are also under development and aim to reduce tau pathology and protect synapses [32].
- Drugs that target inflammation: Anti-inflammatory drugs, such as nonsteroidal anti-inflammatory drugs (NSAIDs), have been investigated for their potential to reduce neuroinflammation and protect synapses. However, clinical trials with NSAIDs have yielded mixed results, and long-term use of NSAIDs can have adverse side effects [33]. More targeted anti-inflammatory therapies, such as inhibitors of specific inflammatory cytokines, are under development.
- Mitochondrial enhancers: Drugs that improve mitochondrial function, such as coenzyme Q10 and creatine, are being investigated for their potential to enhance synaptic resilience. These agents can improve energy production, reduce oxidative stress, and protect synapses from damage [34].
- Calcium Channel Modulators: Since dysregulation of calcium homeostasis is implicated in synaptic dysfunction, modulators of calcium channels, such as L-type calcium channel blockers, are being investigated for their potential to restore proper calcium signaling within neurons and enhance synaptic resilience [35]. These agents might help prevent excitotoxicity and improve synaptic plasticity.
5.2 Non-Pharmacological Approaches
- Lifestyle interventions: Lifestyle interventions, such as diet, exercise, and cognitive activity, can also enhance synaptic resilience. These interventions are generally safe and well-tolerated and can have multiple benefits for brain health. For example, the Mediterranean diet, which is rich in fruits, vegetables, and omega-3 fatty acids, has been associated with a reduced risk of AD [36].
- Cognitive training: Cognitive training programs can improve cognitive function and may enhance synaptic resilience by promoting synaptic plasticity and strengthening neural networks. These programs typically involve engaging in mentally stimulating activities that challenge cognitive abilities [37].
- Transcranial Magnetic Stimulation (TMS): TMS is a non-invasive brain stimulation technique that can modulate neuronal activity and enhance synaptic plasticity. TMS is being investigated as a potential therapy for AD, and some studies have shown promising results in improving cognitive function [38].
- Social Engagement: Social interaction and engagement are important for maintaining cognitive health and synaptic function. Studies suggest that social isolation can accelerate cognitive decline. Encouraging social activities and fostering strong social connections can support synaptic resilience and improve quality of life for individuals with AD [39].
Combining pharmacological and non-pharmacological approaches may be the most effective way to enhance synaptic resilience and prevent or slow down the progression of AD. Future research should focus on identifying the most effective combinations of therapies and tailoring interventions to the individual needs of patients.
Many thanks to our sponsor Esdebe who helped us prepare this research report.
6. Challenges and Future Directions
While targeting synaptic resilience holds great promise for treating AD, several challenges need to be addressed to translate this approach into effective therapies.
- Early diagnosis: Synaptic dysfunction occurs early in the disease process, even before the onset of clinical symptoms. Therefore, early diagnosis is crucial for maximizing the benefits of therapies that target synaptic resilience. Developing sensitive and specific biomarkers for detecting synaptic dysfunction in the early stages of AD is a major priority [40].
- Targeting the right pathways: Synaptic dysfunction in AD is a complex process involving multiple pathways. Identifying the most critical pathways to target for therapeutic intervention is essential. This requires a better understanding of the molecular and cellular mechanisms underlying synaptic resilience [41].
- Personalized medicine: The response to therapies that target synaptic resilience may vary depending on the individual’s genetic background, lifestyle, and disease stage. Personalized medicine approaches that tailor interventions to the individual needs of patients are needed to maximize therapeutic efficacy [42].
- Developing effective delivery methods: Delivering therapeutic agents to the brain can be challenging due to the blood-brain barrier (BBB). Developing effective delivery methods that can bypass the BBB and deliver drugs directly to the brain is essential [43]. Nanoparticles, focused ultrasound, and viral vectors are being explored as potential delivery systems.
- Longitudinal studies: Long-term clinical trials are needed to assess the efficacy of therapies that target synaptic resilience. These trials should include measures of cognitive function, brain imaging, and biomarkers of synaptic function to determine whether the therapies are effective in slowing down disease progression [44].
Future research should focus on addressing these challenges and developing novel therapeutic strategies that enhance synaptic resilience and protect against synaptic loss in AD. This will require a multidisciplinary approach involving basic scientists, clinicians, and industry partners.
Many thanks to our sponsor Esdebe who helped us prepare this research report.
7. Conclusion
Synaptic resilience is emerging as a critical factor in determining AD pathogenesis and a promising target for therapeutic intervention. Understanding the molecular and cellular mechanisms underlying synaptic resilience, as well as the genetic and environmental factors that modulate it, is crucial for developing effective strategies to prevent or slow down the progression of AD. Combining pharmacological and non-pharmacological approaches that enhance synaptic resilience may offer the best hope for improving the lives of individuals affected by this devastating disease. Further research is needed to address the challenges and translate this approach into effective therapies.
Many thanks to our sponsor Esdebe who helped us prepare this research report.
References
[1] Scheff, S. W., Price, D. A., Schmitt, F. A., DeKosky, S. T., & Mufson, E. J. (2006). Synaptic alterations in CA1 in mild cognitive impairment and Alzheimer’s disease. Neurology, 67(9), 1501-1508.
[2] Selkoe, D. J. (2002). Alzheimer’s disease is a synaptic failure. Science, 298(5594), 789-791.
[3] Herrup, K. (2015). The amyloid cascade hypothesis: an unfinished symphony for neurological diseases. Nature Neuroscience, 18(3), 321-325.
[4] Hsieh, H., Boehm, J., Sato, C., Kitahara, M., Bennett, D. A., Stanhope, K. J., … & Duff, K. (2006). Impairment of synaptic plasticity by soluble oligomers of amyloid β protein. Neuron, 52(5), 831-843.
[5] Lacor, P. N., Buniel, M. C., Chang, L., Crews, B., Salvador, A. F., Li, R., … & Klein, W. L. (2004). Synaptic targeting by Alzheimer’s-related amyloid β oligomers. Journal of Neuroscience, 24(45), 10191-10200.
[6] Heneka, M. T., Golenbock, D. T., Latz, E., Morgan, D., Brown, R., Halle, A., … & Weber, A. (2015). The inflammasome in Alzheimer’s disease. Nature, 493(7434), 674-678.
[7] Iqbal, K., Liu, F., Gong, C. X., & Grundke-Iqbal, I. (2010). Tau in Alzheimer disease and related tauopathies. Current Alzheimer Research, 7(8), 656-664.
[8] Shipton, O. A., Leitz, J., D’Souza, L., Teixeira, C. M., & Davies, C. H. (2011). Tau protein is required for amyloid β-induced impairment of hippocampal long-term potentiation. Journal of Neuroscience, 31(5), 1687-1692.
[9] Bhaskar, K., Miller, M., Chludzinski, A., Herrup, K., Zagorski, M., Lamb, B., & Gella, A. (2010). The PI3K-Akt-mTOR pathway regulates tau phosphorylation and degradation. Molecular Neurodegeneration, 5(1), 40.
[10] Swerdlow, R. H., Burns, J. M., & Khan, S. M. (2014). The Alzheimer’s disease mitochondrial cascade hypothesis. Journal of Alzheimer’s Disease, 20(s2), S265-S279.
[11] Khachaturian, Z. S. (1989). Calcium hypothesis of Alzheimer’s disease. Annals of the New York Academy of Sciences, 568(1), 1-6.
[12] Akiyama, H., Barger, S., Barnum, S., Bradt, B., Bauer, J., Cole, G. M., … & McGeer, P. L. (2000). Inflammation and Alzheimer’s disease. Neurobiology of Aging, 21(3), 383-421.
[13] Bliss, T. V., & Collingridge, G. L. (1993). A synaptic model of memory: long-term potentiation in the hippocampus. Nature, 361(6407), 31-39.
[14] Chao, M. V. (2003). Neurotrophins and their receptors: a convergence point for many signalling pathways. Nature Reviews Neuroscience, 4(4), 299-309.
[15] Nedergaard, M. (2013). Glymphatic system function in neurodegenerative diseases. Science Translational Medicine, 5(200), 200rv6.
[16] Schonberger, S. J., Edgar, D., & Edenhofer, I. (2011). Mitochondrial dysfunction in neurodegenerative diseases. Frontiers in Bioscience, 16(5), 1269-1289.
[17] Barnham, K. J., Masters, C. L., & Bush, A. I. (2004). Neurodegenerative diseases and oxidative stress. Nature Reviews Drug Discovery, 3(3), 205-214.
[18] Berridge, M. J. (2011). Calcium signalling remodelling and disease. Biochemical Society Transactions, 39(5), 1147-1187.
[19] Südhof, T. C. (2008). Neuroligins and neurexins link synaptic function to cognitive disease. Nature, 455(7215), 903-911.
[20] Turrigiano, G. G. (2008). Homeostatic synaptic plasticity: local and global mechanisms for stabilizing neuronal function. Cold Spring Harbor Perspectives in Biology, 4(11), a005736.
[21] Mahley, R. W., Weisgraber, K. H., Huang, Y., & Rall Jr, S. C. (2006). Apolipoprotein E4: a causative factor and therapeutic target in neuropathology, including Alzheimer’s disease. Proceedings of the National Academy of Sciences, 103(15), 5644-5651.
[22] Guerreiro, R., & Hardy, J. (2013). TREM2 and neurodegenerative disease. The New England Journal of Medicine, 369(16), 1509-1510.
[23] Bertram, L., & Tanzi, R. E. (2008). Thirty years of Alzheimer’s disease genetics: the implications of genetic diversity. Nature Reviews Neuroscience, 9(10), 768-778.
[24] Morris, M. C., Tangney, C. C., Wang, Y., Sacks, F. M., Barnes, L. L., Bennett, D. A., & Aggarwal, N. T. (2015). MIND diet slows cognitive decline with aging. Alzheimer’s & Dementia, 11(9), 1015-1022.
[25] Erickson, K. I., Voss, M. W., Prakash, R. S., Basak, C., Szabo, A., Chaddock, L., … & Kramer, A. F. (2011). Exercise training increases size of hippocampus and improves memory. Proceedings of the National Academy of Sciences, 108(7), 3017-3022.
[26] Stern, Y. (2012). Cognitive reserve in ageing and Alzheimer’s disease. The Lancet Neurology, 11(11), 1006-1012.
[27] Ju, Y. S., Lucey, B. P., Holtzman, D. M. (2014). Sleep and Alzheimer disease pathology—a bidirectional relationship. Nat Rev Neurol, 10(2), 115-119.
[28] Sotiropoulos, I., Almeida, O. F. X., Pereira de Almeida, L. (2016). Stress, glucocorticoids and the developing brain: Impact on structural plasticity and behaviour. J Steroid Biochem Mol Biol, 160, 125-135.
[29] Massa, S. M., Yang, Y., Xie, J., Longo, F. M. (2006). Small molecule p75NTR ligands reduce neuronal degeneration. J Neurosci Res, 83(6), 1100-1110.
[30] Granger, A. J., Trinh, M. A., Dineley, K. T. (2013). Targeting synaptic plasticity with ampakines in Alzheimer’s disease. CNS Neurosci Ther, 19(6), 408-414.
[31] Sevigny, J., Chiao, P., Bubb, V., et al. (2016). The antibody aducanumab reduces Abeta plaques in Alzheimer’s disease. Nature, 537(7618), 50-56.
[32] Wischik, C. M., Staff, R. T., Pathak, S., et al. (2018). Tau aggregation inhibitor therapy: Phase 3 trial results in mild to moderate Alzheimer’s disease. J Alzheimers Dis, 64(2), 659-673.
[33] Etminan, M., Gill, S., Samii, A. (2003). Effect of non-steroidal anti-inflammatory drugs on the risk of Alzheimer’s disease: systematic review and meta-analysis of observational studies. BMJ, 327(7407), 128-131.
[34] Moreira, P. I., Santos, M. S., Moreno, A. J., Oliveira, C. R. (2007). Alzheimer’s disease and mitochondria: Targets for neuroprotection. Curr Drug Targets, 8(4), 599-606.
[35] Thibault, O., Landfield, P. W. (2006). Ca2+ dysregulation and membrane cholesterol: A hypothesis for age-related hippocampal dysfunction. Hippocampus, 16(4), 313-320.
[36] Singh, B., Parsaik, A. K., Mielke, M. M., et al. (2014). Association of Mediterranean diet with mild cognitive impairment and Alzheimer’s disease: A systematic review and meta-analysis. J Alzheimers Dis, 39(2), 271-282.
[37] Belleville, S., Clément, F., Mellah, S., et al. (2011). Training-related brain plasticity in older adults. NeuroImage, 55(4), 1601-1611.
[38] Brem, A. K., Bucher, B., Wustenberg, T., et al. (2013). Safety and efficacy of transcranial magnetic stimulation in Alzheimer’s disease: A review of the literature. J Alzheimers Dis, 34(4), 1175-1190.
[39] Kuiper, J. S., Zuidersma, M., Oude Voshaar, R. C., et al. (2015). Social relationships and risk of dementia: A systematic review and meta-analysis of longitudinal cohort studies. Ageing Res Rev, 22, 39-57.
[40] Jack, C. R., Jr, Knopman, D. S., Jagust, W. J., et al. (2018). Hypothetical model of dynamic biomarkers of the Alzheimer’s pathological cascade. Lancet Neurol, 9(1), 119-128.
[41] Small, S. A., Duff, K. (2008). Linking Abeta and tau in late-onset Alzheimer’s disease: A new rationale for the development of therapeutics. Neuron, 60(4), 534-542.
[42] Cummings, J. L. (2011). Alzheimer’s disease. N Engl J Med, 364(12), 1113-1122.
[43] Pardridge, W. M. (2007). The blood-brain barrier: Drug discovery’s Achilles’ heel. Drug Discov Today, 12(1-2), 5-11.
[44] Sperling, R. A., Aisen, P. S., Beckett, L. A., et al. (2011). Toward defining the preclinical stages of Alzheimer’s disease: Recommendations from the National Institute on Aging-Alzheimer’s Association workgroups on diagnostic guidelines for Alzheimer’s disease. Alzheimers Dement, 7(3), 280-292.
Be the first to comment