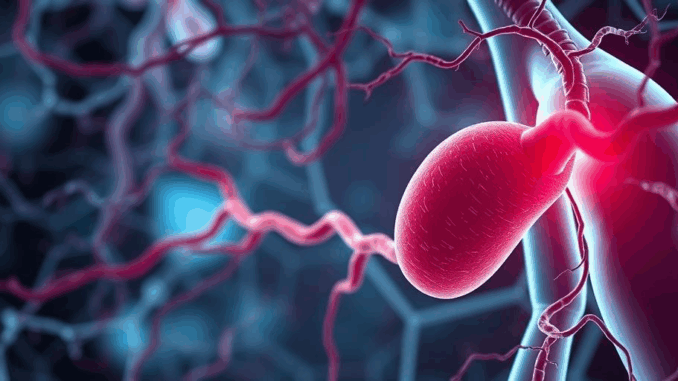
Abstract
The arterial system, a complex network of vessels responsible for oxygen and nutrient delivery, is subject to a multitude of pathological conditions, ranging from atherosclerosis and aneurysm formation to dissection and vascular malformations. While significant progress has been made in understanding the pathophysiology and treatment of these diseases, several challenges remain, particularly concerning the long-term efficacy of interventions and the development of strategies for arterial regeneration. This report provides a comprehensive overview of the arterial system, delving into its intricate structure and function, prevalent diseases, diagnostic modalities, and therapeutic approaches. Furthermore, it explores cutting-edge research in mechanobiology, focusing on how mechanical forces influence arterial remodeling and disease progression. Finally, it examines the promising field of arterial regeneration, highlighting recent advances in stem cell therapies, biomaterial engineering, and the development of bioartificial vessels. The report aims to provide a critical assessment of the current state of knowledge and identify key areas for future research to advance our understanding and treatment of arterial diseases.
Many thanks to our sponsor Esdebe who helped us prepare this research report.
1. Introduction
The arterial system is a hierarchical network of blood vessels that transports oxygenated blood and nutrients from the heart to the tissues and organs throughout the body. This intricate system comprises large elastic arteries, muscular arteries, and arterioles, each with distinct structural and functional characteristics. The aorta, the largest artery, originates from the left ventricle and branches into major arteries that supply different regions of the body. These arteries further divide into smaller arterioles, which regulate blood flow to the capillaries, the smallest blood vessels where oxygen and nutrient exchange occurs. The structural integrity and functional properties of the arterial wall are crucial for maintaining proper blood pressure, regulating blood flow, and preventing vascular diseases.
The arterial wall is composed of three distinct layers: the tunica intima, tunica media, and tunica adventitia. The tunica intima, the innermost layer, consists of a single layer of endothelial cells (ECs) that line the lumen. These ECs play a critical role in regulating vascular tone, permeability, and inflammation. The tunica media, the middle layer, is composed of smooth muscle cells (SMCs) and elastic fibers. SMCs are responsible for vasoconstriction and vasodilation, which control blood flow and blood pressure. Elastic fibers provide elasticity and recoil to the arterial wall, allowing it to withstand the pulsatile pressure generated by the heart. The tunica adventitia, the outermost layer, consists of connective tissue, collagen fibers, and vasa vasorum, small blood vessels that supply the arterial wall itself. The adventitia provides structural support and anchors the artery to surrounding tissues.
Arterial diseases, such as atherosclerosis, aneurysm, and dissection, are major causes of morbidity and mortality worldwide. Atherosclerosis, characterized by the formation of plaques in the arterial wall, is a leading cause of heart disease, stroke, and peripheral artery disease. Aneurysms, characterized by localized dilation of the arterial wall, can rupture and cause life-threatening hemorrhage. Dissections, characterized by a tear in the intima, can lead to blood entering the arterial wall and potentially occluding blood flow. Understanding the underlying mechanisms of these diseases is crucial for developing effective prevention and treatment strategies.
Many thanks to our sponsor Esdebe who helped us prepare this research report.
2. Arterial Structure and Function
2.1. The Endothelium: A Multifunctional Interface
The endothelium, a monolayer of specialized epithelial cells lining the inner surface of all blood vessels, is far more than a simple barrier. It actively regulates vascular tone, permeability, inflammation, and thrombosis, playing a pivotal role in maintaining vascular homeostasis. Endothelial cells (ECs) respond to a variety of stimuli, including shear stress, growth factors, and inflammatory cytokines, modulating the expression of genes involved in these processes.
One of the key functions of the endothelium is the production of vasoactive substances, such as nitric oxide (NO) and endothelin-1 (ET-1). NO, a potent vasodilator, is synthesized by endothelial nitric oxide synthase (eNOS) in response to shear stress and other stimuli. NO diffuses into the underlying smooth muscle cells, causing relaxation and vasodilation. ET-1, a potent vasoconstrictor, is produced by ECs in response to various stimuli, including angiotensin II and thrombin. The balance between NO and ET-1 regulates vascular tone and blood pressure. Endothelial dysfunction, characterized by impaired NO production and increased ET-1 production, is a hallmark of many cardiovascular diseases, including atherosclerosis, hypertension, and diabetes.
Furthermore, the endothelium regulates vascular permeability, controlling the passage of molecules and cells across the vessel wall. ECs form tight junctions that restrict the passage of large molecules, while specialized transport mechanisms allow for the selective transport of specific substances. Inflammatory cytokines, such as tumor necrosis factor-alpha (TNF-α) and interleukin-1β (IL-1β), can disrupt endothelial barrier function, leading to increased permeability and edema. The endothelium also plays a critical role in regulating inflammation by expressing adhesion molecules, such as ICAM-1 and VCAM-1, which promote leukocyte adhesion and transmigration into the vessel wall. This process is crucial for immune surveillance and wound healing but can also contribute to the development of inflammatory diseases like atherosclerosis.
2.2. Smooth Muscle Cells: Contractility and Extracellular Matrix Remodeling
The tunica media, the middle layer of the arterial wall, is primarily composed of smooth muscle cells (SMCs) and extracellular matrix (ECM). SMCs are responsible for regulating vascular tone through contraction and relaxation, thereby controlling blood flow and blood pressure. In addition to their contractile function, SMCs also play a crucial role in ECM remodeling, synthesizing and degrading various ECM components, such as collagen, elastin, and proteoglycans. This dynamic process is essential for maintaining the structural integrity and mechanical properties of the arterial wall.
SMC contraction is regulated by a complex interplay of intracellular signaling pathways, including calcium signaling, myosin light chain phosphorylation, and Rho kinase activation. Various stimuli, such as vasoactive substances, neurotransmitters, and mechanical forces, can trigger SMC contraction or relaxation. In response to increased intracellular calcium levels, myosin light chain kinase (MLCK) phosphorylates myosin light chain (MLC), leading to the formation of cross-bridges between actin and myosin filaments and SMC contraction. Rho kinase, activated by various stimuli, inhibits myosin light chain phosphatase (MLCP), an enzyme that dephosphorylates MLC, thereby maintaining SMC contraction. SMC relaxation is mediated by decreased intracellular calcium levels and activation of MLCP.
SMCs also play a critical role in ECM remodeling, synthesizing and secreting various ECM components, including collagen, elastin, and proteoglycans. These ECM components provide structural support and elasticity to the arterial wall. Collagen fibers provide tensile strength, while elastin fibers provide recoil. Proteoglycans regulate water content and facilitate cell-matrix interactions. SMCs also produce matrix metalloproteinases (MMPs), enzymes that degrade ECM components. MMP activity is tightly regulated by tissue inhibitors of metalloproteinases (TIMPs), ensuring a balance between ECM synthesis and degradation. Dysregulation of ECM remodeling, characterized by increased MMP activity and decreased collagen synthesis, can lead to arterial weakening and aneurysm formation.
2.3. The Adventitia: Structural Support and Vasa Vasorum
The tunica adventitia, the outermost layer of the arterial wall, provides structural support and anchors the artery to surrounding tissues. It is composed of connective tissue, collagen fibers, and vasa vasorum, small blood vessels that supply the arterial wall itself. The adventitia plays a critical role in regulating inflammation and adventitial remodeling in response to vascular injury and disease.
The adventitia is rich in collagen fibers, which provide tensile strength and prevent excessive dilation of the artery. Fibroblasts, the primary cell type in the adventitia, synthesize and secrete collagen fibers. The adventitia also contains various other cell types, including immune cells, such as macrophages and T cells, which play a role in inflammation. Vasa vasorum, small blood vessels that supply the arterial wall, are particularly abundant in the adventitia of large arteries. These vessels provide oxygen and nutrients to the outer layers of the arterial wall, as diffusion from the lumen is insufficient to meet the metabolic demands of these layers.
Adventitial remodeling, characterized by increased collagen deposition, inflammation, and neovascularization, is a common feature of arterial diseases, such as atherosclerosis and aneurysm. Inflammation in the adventitia can promote the recruitment of immune cells and the production of inflammatory cytokines, which contribute to vascular dysfunction. Neovascularization, the formation of new blood vessels in the adventitia, can promote plaque growth and aneurysm expansion. Targeting adventitial remodeling may be a promising therapeutic strategy for preventing and treating arterial diseases.
Many thanks to our sponsor Esdebe who helped us prepare this research report.
3. Arterial Diseases: Pathophysiology and Clinical Manifestations
3.1. Atherosclerosis: Plaque Formation and Vascular Obstruction
Atherosclerosis is a chronic inflammatory disease characterized by the formation of plaques in the arterial wall. These plaques are composed of lipids, inflammatory cells, and extracellular matrix. Atherosclerosis is a leading cause of heart disease, stroke, and peripheral artery disease.
The pathogenesis of atherosclerosis involves a complex interplay of risk factors, including hyperlipidemia, hypertension, smoking, and diabetes. These risk factors damage the endothelium, leading to endothelial dysfunction. Damaged endothelium becomes more permeable to lipoproteins, such as low-density lipoprotein (LDL), which accumulate in the arterial wall. Accumulated LDL undergoes oxidation, forming oxidized LDL (oxLDL). OxLDL is recognized by macrophages, which engulf it and become foam cells. Foam cells accumulate in the arterial wall, forming fatty streaks, the earliest lesion of atherosclerosis. As atherosclerosis progresses, foam cells die and release lipids, which accumulate in the extracellular space, forming a lipid core. The lipid core is covered by a fibrous cap, composed of smooth muscle cells and collagen fibers. The fibrous cap provides structural stability to the plaque. However, the fibrous cap can rupture, exposing the lipid core to the bloodstream. This triggers thrombus formation, which can occlude the artery and cause acute ischemic events, such as myocardial infarction or stroke.
3.2. Aneurysms: Wall Weakening and Rupture Risk
An aneurysm is a localized dilation of the arterial wall. Aneurysms can occur in any artery but are most common in the aorta, brain, and peripheral arteries. Aneurysms can be caused by various factors, including genetic predisposition, hypertension, atherosclerosis, and infection. Aneurysms pose a significant risk of rupture, which can lead to life-threatening hemorrhage. The risk of rupture is related to the size and growth rate of the aneurysm.
3.3. Arterial Dissection: Intimal Tears and Hematoma Formation
Arterial dissection is a condition characterized by a tear in the intima, the innermost layer of the arterial wall. This allows blood to enter the arterial wall, forming a hematoma that can propagate along the artery. Arterial dissection can occur in any artery but is most common in the aorta and carotid arteries. Arterial dissection can be caused by various factors, including hypertension, connective tissue disorders, and trauma. Arterial dissection can lead to various complications, including ischemia, stroke, and rupture.
Many thanks to our sponsor Esdebe who helped us prepare this research report.
4. Diagnostic Techniques for Arterial Diseases
4.1. Angiography: Visualizing Arterial Anatomy
Angiography is an imaging technique that uses X-rays and contrast dye to visualize the arteries. Angiography is used to diagnose various arterial diseases, including atherosclerosis, aneurysms, and dissections. During angiography, a catheter is inserted into an artery, and contrast dye is injected. X-rays are then taken to visualize the arteries. Angiography can provide detailed information about the size, shape, and location of arterial lesions.
4.2. Ultrasound: Non-Invasive Arterial Imaging
Ultrasound is a non-invasive imaging technique that uses sound waves to visualize the arteries. Ultrasound is used to diagnose various arterial diseases, including atherosclerosis, aneurysms, and dissections. During ultrasound, a transducer is placed on the skin over the artery, and sound waves are emitted. The sound waves are reflected back to the transducer, and an image of the artery is created. Ultrasound can provide information about the size, shape, and blood flow velocity in the artery.
4.3. Computed Tomography Angiography (CTA) and Magnetic Resonance Angiography (MRA)
CTA and MRA are advanced imaging techniques that provide detailed three-dimensional images of the arteries. CTA uses X-rays and contrast dye, while MRA uses magnetic fields and radio waves. These techniques are used to diagnose various arterial diseases, including atherosclerosis, aneurysms, and dissections. CTA and MRA can provide information about the size, shape, location, and composition of arterial lesions.
Many thanks to our sponsor Esdebe who helped us prepare this research report.
5. Medical and Surgical Treatments for Arterial Conditions
5.1. Pharmacological Management of Arterial Diseases
Pharmacological management plays a crucial role in the prevention and treatment of arterial diseases. Medications commonly used include antiplatelet agents (e.g., aspirin, clopidogrel) to prevent thrombus formation, statins to lower cholesterol levels, antihypertensive drugs to control blood pressure, and vasodilators to improve blood flow. These medications can reduce the risk of cardiovascular events, such as heart attack and stroke, and improve the quality of life for patients with arterial diseases.
5.2. Endovascular Interventions: Minimally Invasive Procedures
Endovascular interventions are minimally invasive procedures that are used to treat various arterial diseases. These procedures are performed through small incisions or punctures in the skin, using catheters and specialized devices. Common endovascular interventions include angioplasty, stenting, and coil embolization. Angioplasty involves inflating a balloon inside a narrowed artery to widen it. Stenting involves placing a metal mesh tube (stent) inside the artery to keep it open. Coil embolization involves placing coils inside an aneurysm to block blood flow and prevent rupture. Endovascular interventions offer several advantages over open surgery, including smaller incisions, shorter hospital stays, and faster recovery times.
5.3. Open Surgical Repair: Traditional Approaches for Complex Cases
Open surgical repair is a traditional approach for treating complex arterial diseases. This involves making a large incision to access the affected artery and performing surgical procedures to repair or replace the damaged vessel. Common open surgical procedures include bypass grafting, aneurysm repair, and endarterectomy. Bypass grafting involves creating a new pathway for blood flow around a blocked artery. Aneurysm repair involves replacing the weakened artery with a graft. Endarterectomy involves removing plaque from the inside of an artery. Open surgical repair is typically reserved for cases where endovascular interventions are not feasible or have failed.
Many thanks to our sponsor Esdebe who helped us prepare this research report.
6. Mechanobiology of Arterial Remodeling
6.1. Shear Stress and Arterial Endothelial Function
Mechanical forces, particularly shear stress exerted by blood flow on the endothelium, play a critical role in regulating arterial remodeling and disease progression. Shear stress is the frictional force exerted by flowing blood on the inner surface of the artery. Endothelial cells (ECs) are highly sensitive to shear stress and respond by modulating their gene expression, morphology, and function. Physiological levels of shear stress promote endothelial cell alignment, NO production, and anti-inflammatory signaling. In contrast, disturbed or low shear stress promotes endothelial dysfunction, inflammation, and atherosclerosis. Furthermore, oscillatory shear stress or regions of flow separation are often associated with plaque development. Specific mechanosensors, such as PECAM-1, VEGFR2, and integrins, mediate the EC response to shear stress, initiating intracellular signaling cascades that regulate gene expression and cell behavior. Understanding the mechanotransduction pathways involved in shear stress-mediated endothelial function is crucial for developing targeted therapies to prevent and treat arterial diseases. A deeper understanding of disturbed flow patterns and their impact on the endothelium could significantly improve risk stratification and intervention strategies.
6.2. Cyclic Strain and Smooth Muscle Cell Behavior
Cyclic strain, the repetitive stretching and relaxation of the arterial wall caused by pulsatile blood pressure, is another important mechanical force that influences arterial remodeling. Smooth muscle cells (SMCs) in the tunica media are constantly subjected to cyclic strain, which regulates their proliferation, differentiation, and ECM production. Physiological levels of cyclic strain promote SMC quiescence and maintain the structural integrity of the arterial wall. In contrast, excessive or abnormal cyclic strain can induce SMC proliferation, migration, and ECM remodeling, contributing to arterial stiffening, aneurysm formation, and other vascular diseases. The underlying mechanisms of cyclic strain-mediated SMC behavior involve various signaling pathways, including integrin signaling, MAPK activation, and Rho kinase activation. Targeting these pathways may offer potential therapeutic strategies for preventing and treating arterial diseases associated with abnormal cyclic strain. Indeed, understanding the impact of different strain magnitudes and frequencies is critical for designing effective interventions that normalize arterial wall mechanics.
6.3. The Role of Extracellular Matrix in Arterial Mechanics
The extracellular matrix (ECM) plays a crucial role in determining the mechanical properties of the arterial wall. The ECM is composed of various proteins, including collagen, elastin, and proteoglycans, which provide structural support, elasticity, and tensile strength to the artery. The composition and organization of the ECM are dynamically regulated by SMCs in response to mechanical forces and other stimuli. Alterations in ECM composition, such as increased collagen deposition or elastin degradation, can lead to arterial stiffening, aneurysm formation, and other vascular diseases. MMPs, a family of enzymes that degrade ECM components, play a critical role in ECM remodeling. Imbalances between MMP activity and TIMP expression can contribute to arterial weakening and aneurysm expansion. Targeting ECM remodeling may be a promising therapeutic strategy for preventing and treating arterial diseases. The interplay between ECM components and cell-ECM interactions is complex, and future research should focus on unraveling the intricate mechanisms involved in ECM remodeling in arterial diseases. In particular, the role of glycosaminoglycans (GAGs) and their sulfation patterns warrants further investigation.
Many thanks to our sponsor Esdebe who helped us prepare this research report.
7. Arterial Regeneration: Emerging Therapies
7.1. Stem Cell Therapies for Arterial Repair
Stem cell therapies hold great promise for arterial repair and regeneration. Various types of stem cells, including embryonic stem cells (ESCs), induced pluripotent stem cells (iPSCs), and adult stem cells (ASCs), have been investigated for their potential to differentiate into endothelial cells (ECs) and smooth muscle cells (SMCs) and promote vascular regeneration. ESCs and iPSCs have the potential to differentiate into any cell type in the body, including ECs and SMCs. However, their use is limited by ethical concerns and the risk of teratoma formation. ASCs, such as bone marrow-derived mesenchymal stem cells (MSCs) and endothelial progenitor cells (EPCs), are more readily available and have a lower risk of teratoma formation. MSCs can secrete growth factors and cytokines that promote angiogenesis and vascular remodeling. EPCs can differentiate into ECs and contribute to endothelial repair. Clinical trials have shown that stem cell therapies can improve blood flow and reduce limb amputation rates in patients with peripheral artery disease. However, the long-term efficacy and safety of stem cell therapies for arterial repair remain to be fully established. The challenge lies in optimizing stem cell delivery, survival, and differentiation within the damaged arterial environment. The use of biomaterial scaffolds to support stem cell engraftment and promote vascular regeneration is an area of intense research.
7.2. Biomaterial Scaffolds for Vascular Engineering
Biomaterial scaffolds provide a structural support for cell growth and tissue regeneration. Various types of biomaterials, including natural polymers (e.g., collagen, elastin, alginate) and synthetic polymers (e.g., polyglycolic acid, polylactic acid, polycaprolactone), have been used to fabricate vascular scaffolds. Natural polymers are biocompatible and biodegradable but may lack the mechanical strength required for vascular applications. Synthetic polymers can be tailored to have specific mechanical properties and degradation rates. Vascular scaffolds can be seeded with cells, such as ECs and SMCs, to create bioartificial vessels. These bioartificial vessels can be implanted into the body to replace damaged arteries. Decellularized arteries, which are arteries from which all cells have been removed, can also be used as vascular scaffolds. Decellularized arteries retain the native ECM structure and provide a natural environment for cell growth and tissue regeneration. However, the decellularization process can damage the ECM, affecting the mechanical properties of the scaffold. Optimizing the decellularization process and crosslinking the ECM may improve the performance of decellularized arterial scaffolds. Additive manufacturing techniques, such as 3D printing, are emerging as powerful tools for fabricating complex and customized vascular scaffolds. These techniques allow for precise control over scaffold architecture, porosity, and mechanical properties. Future research should focus on developing novel biomaterials and fabrication techniques to create vascular scaffolds that promote rapid and complete arterial regeneration. The integration of growth factors and other bioactive molecules into the scaffold can further enhance cell adhesion, proliferation, and differentiation.
7.3. Bioartificial Vessels: A Promising Alternative
Bioartificial vessels, engineered blood vessels created in the laboratory, represent a promising alternative to synthetic grafts for arterial replacement. These vessels are typically constructed by seeding cells, such as ECs and SMCs, onto a biomaterial scaffold. The cells proliferate and deposit ECM, forming a functional blood vessel. Bioartificial vessels offer several advantages over synthetic grafts, including improved biocompatibility, reduced risk of thrombosis, and potential for growth and remodeling. Several strategies have been developed to create bioartificial vessels, including tissue engineering, self-assembly, and decellularization. Tissue engineering involves seeding cells onto a biodegradable scaffold and culturing them in a bioreactor to promote tissue formation. Self-assembly involves using cells that spontaneously organize into a vascular structure. Decellularization involves removing all cells from a native blood vessel, leaving behind the ECM scaffold. The ECM scaffold can then be seeded with cells to create a bioartificial vessel. Clinical trials have shown that bioartificial vessels can successfully replace damaged arteries in patients with peripheral artery disease and congenital heart defects. However, the long-term patency and performance of bioartificial vessels remain to be further evaluated. Scalability, cost-effectiveness, and the ability to create vessels with appropriate mechanical properties remain significant challenges. The development of fully autologous bioartificial vessels, created from the patient’s own cells, could further improve biocompatibility and reduce the risk of rejection.
Many thanks to our sponsor Esdebe who helped us prepare this research report.
8. Conclusion
The arterial system is a complex and dynamic network of vessels that plays a critical role in maintaining overall health. Arterial diseases, such as atherosclerosis, aneurysm, and dissection, are major causes of morbidity and mortality worldwide. While significant progress has been made in understanding the pathophysiology and treatment of these diseases, several challenges remain. Emerging research in mechanobiology and arterial regeneration holds great promise for developing novel therapies to prevent and treat arterial diseases. A deeper understanding of the mechanical forces that influence arterial remodeling, the development of advanced biomaterials for vascular engineering, and the utilization of stem cell therapies for arterial repair may lead to more effective and durable treatments for arterial diseases in the future. In particular, a focus on personalized medicine, tailoring treatments to individual patient characteristics and disease phenotypes, is likely to improve outcomes. Furthermore, preventative strategies, focusing on lifestyle modifications and early detection of risk factors, are essential for reducing the burden of arterial diseases.
Many thanks to our sponsor Esdebe who helped us prepare this research report.
References
- Ross, R. (1993). The pathogenesis of atherosclerosis: a perspective for the 1990s. Nature, 362(6423), 801-809.
- Raffetto, J. D., & Khalil, R. A. (2008). Mechanisms of venous valve incompetence: implications for the pathogenesis of varicose veins. Journal of Vascular Surgery, 47(1), 218-229.
- Erbel, R., Di Cesare, E., Bartels, C., & Kahlert, P. (2014). Aortic aneurysm. Heart, 100(21), 1637-1644.
- Hutchinson, S., & Eagle, K. A. (2008). Acute aortic dissection. Circulation, 118(25), 2754-2779.
- Davies, P. F. (1995). Flow-mediated endothelial mechanotransduction. Physiological Reviews, 75(3), 519-560.
- Humphrey, J. D. (2002). Cardiovascular solid mechanics: cells, tissues, and organs. Springer Science & Business Media.
- L’Heureux, N., McAllister, T. N., & de la Fuente, L. M. (2006). Tissue-engineered blood vessel for adult arterial replacement. New England Journal of Medicine, 355(6), 573-580.
- Nerem, R. M., & Girard, P. R. (1990). Hemodynamic influences on vascular endothelial biology. Toxicologic Pathology, 18(4), 572-582.
- Chien, S. (2008). Mechanotransduction and endothelial cell homeostasis: the wisdom within. American Journal of Physiology-Heart and Circulatory Physiology, 295(1), H1-H18.
- Owens, G. K., Kumar, M. S., & Wamhoff, B. R. (2004). Molecular regulation of vascular smooth muscle cell differentiation and phenotypic modulation. Physiological Reviews, 84(3), 767-801.
- Carmeliet, P. (2000). Mechanisms of angiogenesis and arteriogenesis. Nature medicine, 6(4), 389-395.
- Dzau, V. J., & Gnecchi, M. (2008). Therapeutic potential of endothelial progenitor cells in cardiovascular disease. Circulation, 118(10), 987-992.
- Badylak, S. F., Freytes, D. O., & Gilbert, T. W. (2009). Extracellular matrix as a biological scaffold material: structure and function. Acta biomaterialia, 5(1), 1-13.
- Atala, A., Murphy, S. V., & Yoo, J. J. (2014). 3D bioprinting in regenerative medicine. Advanced Drug Delivery Reviews, 79, 2-17.
- Taylor, D. A. (2013). Bioartificial organs: moving toward clinical reality. Expert Review of Medical Devices, 10(4), 483-492.
- Tabas, I., García-Cardeña, G., & Owens, G. K. (2015). Cellular mechanisms of atherosclerosis. Journal of the American College of Cardiology, 66(14), 1690-1703.
Considering the challenges in stem cell delivery and survival for arterial repair, how might advancements in nanotechnology, specifically nanoparticles for targeted drug release, improve the efficacy of these therapies by protecting and guiding stem cells to the damaged arterial tissue?
That’s a great point! Nanoparticle-mediated delivery is definitely a promising avenue. Protecting stem cells and ensuring targeted delivery to damaged tissue using nanotechnology could significantly enhance the effectiveness of arterial regeneration therapies. This could reduce off-target effects and improve overall outcomes. Exciting possibilities!
Editor: MedTechNews.Uk
Thank you to our Sponsor Esdebe
Given the emphasis on mechanobiology, how can computational modeling and in silico simulations enhance our understanding of arterial remodeling under varying hemodynamic conditions, potentially accelerating the development of targeted therapies?