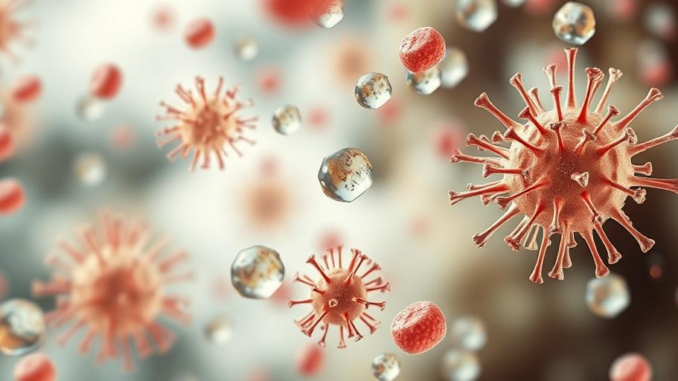
Abstract
The relentless rise of antibiotic resistance poses a critical threat to global health. This report provides a comprehensive overview of the current state of antibiotic resistance, the mechanisms driving its spread, and the strategies being developed to combat this challenge. We delve into the intricacies of antibiotic mechanisms of action and resistance mechanisms, examining both established drug classes and emerging therapeutic approaches. The report also explores the development pipeline for novel antibiotics, focusing on innovative targets and drug delivery systems, and addresses the economic and regulatory hurdles that impede antibiotic innovation. We analyze the importance of antimicrobial stewardship programs in optimizing antibiotic use and preventing the further emergence of resistance. Furthermore, we discuss the potential of alternative therapies, such as phage therapy and immunotherapy, in complementing or replacing traditional antibiotics. Finally, we offer perspectives on the future direction of antibiotic research and development, emphasizing the need for collaborative efforts and sustained investment to ensure the continued efficacy of antimicrobial therapies.
Many thanks to our sponsor Esdebe who helped us prepare this research report.
1. Introduction: The Antibiotic Resistance Crisis
The discovery and widespread use of antibiotics in the 20th century revolutionized medicine, transforming previously deadly infections into readily treatable conditions. However, the remarkable success of antibiotics has been shadowed by the emergence and proliferation of antibiotic-resistant bacteria, a phenomenon that now threatens to undermine the foundations of modern healthcare. Antibiotic resistance occurs when bacteria evolve mechanisms that allow them to survive exposure to antibiotics that would normally kill or inhibit their growth. This resistance can arise through various mechanisms, including genetic mutations, horizontal gene transfer, and adaptive responses to antibiotic exposure.
The consequences of antibiotic resistance are far-reaching. Resistant infections are associated with increased morbidity, mortality, healthcare costs, and hospital stays [1]. The spread of multidrug-resistant organisms (MDROs), such as methicillin-resistant Staphylococcus aureus (MRSA), vancomycin-resistant Enterococci (VRE), and carbapenem-resistant Enterobacteriaceae (CRE), has become a major public health concern. The World Health Organization (WHO) has declared antibiotic resistance one of the top 10 global health threats facing humanity [2].
Urinary tract infections (UTIs) serve as a prime example of the challenges posed by antibiotic resistance. UTIs are among the most common bacterial infections worldwide, and Escherichia coli is the predominant causative agent. However, the increasing prevalence of antibiotic-resistant E. coli strains, particularly those producing extended-spectrum beta-lactamases (ESBLs), has complicated the treatment of UTIs [3]. The emergence of new antibiotics like Pivya (olamine fosfomycin) and Orlynvah (oritavancin), while approved for specific indications, underscores the urgent need for novel therapeutic options to combat resistant UTIs and other infections. While Pivya is used for UTIs Orlynvah is an option for Skin infections. This report addresses the broader context of antibiotic resistance and the development of novel antimicrobial strategies. It aims to provide expert-level insights into the mechanisms, challenges, and future directions in the field of antibiotic research and development.
Many thanks to our sponsor Esdebe who helped us prepare this research report.
2. Mechanisms of Antibiotic Action and Resistance
Antibiotics exert their antimicrobial effects through a variety of mechanisms, targeting essential bacterial processes such as cell wall synthesis, protein synthesis, DNA replication, and metabolic pathways. Understanding these mechanisms is crucial for developing new antibiotics and overcoming resistance.
2.1. Mechanisms of Antibiotic Action
-
Inhibition of Cell Wall Synthesis: Beta-lactam antibiotics (e.g., penicillin, cephalosporins, carbapenems) inhibit the synthesis of peptidoglycan, a critical component of the bacterial cell wall. These antibiotics bind to penicillin-binding proteins (PBPs), enzymes responsible for cross-linking peptidoglycan chains, thereby disrupting cell wall assembly and leading to cell lysis [4]. Glycopeptides (e.g., vancomycin) also inhibit cell wall synthesis, but by a different mechanism. Vancomycin binds to the D-alanyl-D-alanine terminus of peptidoglycan precursors, preventing their incorporation into the cell wall [5].
-
Inhibition of Protein Synthesis: Several classes of antibiotics target bacterial ribosomes, the molecular machines responsible for protein synthesis. Aminoglycosides (e.g., gentamicin, tobramycin) bind to the 30S ribosomal subunit, interfering with mRNA translation and causing misreading of the genetic code [6]. Tetracyclines also bind to the 30S subunit, preventing tRNA from binding to the ribosome [7]. Macrolides (e.g., erythromycin, azithromycin) bind to the 23S rRNA component of the 50S ribosomal subunit, blocking the translocation of tRNA and inhibiting protein synthesis [8].
-
Inhibition of Nucleic Acid Synthesis: Quinolones (e.g., ciprofloxacin, levofloxacin) inhibit DNA replication by targeting bacterial DNA gyrase and topoisomerase IV, enzymes essential for DNA supercoiling and strand separation [9]. Rifamycins (e.g., rifampin) inhibit RNA synthesis by binding to bacterial RNA polymerase, preventing the initiation of transcription [10].
-
Inhibition of Metabolic Pathways: Sulfonamides and trimethoprim inhibit folate synthesis, a critical metabolic pathway in bacteria. Sulfonamides inhibit dihydropteroate synthase, while trimethoprim inhibits dihydrofolate reductase, both enzymes involved in the synthesis of tetrahydrofolic acid, a cofactor essential for nucleotide synthesis [11].
2.2. Mechanisms of Antibiotic Resistance
Bacteria have evolved diverse mechanisms to resist the effects of antibiotics, including:
-
Enzymatic Inactivation: Bacteria can produce enzymes that degrade or modify antibiotics, rendering them inactive. Beta-lactamases are a prime example, hydrolyzing the beta-lactam ring of beta-lactam antibiotics, leading to resistance [12]. Aminoglycoside-modifying enzymes (AMEs) modify aminoglycosides through acetylation, phosphorylation, or adenylation, reducing their affinity for the ribosome [13].
-
Target Modification: Bacteria can alter the target site of an antibiotic, preventing it from binding effectively. Mutations in PBPs can reduce the affinity of beta-lactam antibiotics [14]. Mutations in the 23S rRNA can confer resistance to macrolides [15]. Mutations in DNA gyrase and topoisomerase IV can lead to quinolone resistance [16].
-
Efflux Pumps: Bacteria can express efflux pumps that actively transport antibiotics out of the cell, reducing their intracellular concentration. Multidrug efflux pumps can confer resistance to multiple classes of antibiotics [17].
-
Reduced Permeability: Bacteria can reduce the permeability of their cell membrane, preventing antibiotics from entering the cell. This can occur through mutations in porins, channels that allow antibiotics to pass through the outer membrane of Gram-negative bacteria [18].
-
Target Bypass: Bacteria can develop alternative metabolic pathways that bypass the inhibited target. For example, some bacteria can acquire an alternative dihydrofolate reductase that is resistant to trimethoprim [19].
-
Biofilm Formation: Bacteria can form biofilms, complex communities of cells encased in an extracellular matrix. Biofilms provide a physical barrier that protects bacteria from antibiotics and host immune defenses [20]. Bacteria in biofilms often exhibit increased antibiotic resistance compared to planktonic cells.
Many thanks to our sponsor Esdebe who helped us prepare this research report.
3. The Development Pipeline for Novel Antibiotics
The development of new antibiotics is a complex and challenging process, requiring significant investment in research and development. The antibiotic development pipeline has been relatively stagnant in recent decades, with few new classes of antibiotics reaching the market [21]. This lack of innovation is due to a combination of scientific, economic, and regulatory factors.
3.1. Challenges in Antibiotic Development
-
Scientific Challenges: Identifying novel targets and developing drugs that effectively inhibit these targets while minimizing toxicity to host cells is a significant challenge. Bacteria have evolved sophisticated mechanisms to evade antibiotics, making it difficult to develop drugs that can overcome these resistance mechanisms.
-
Economic Challenges: The development of new antibiotics is often not economically attractive to pharmaceutical companies. Antibiotics are typically used for short durations, and their use is often restricted to prevent the emergence of resistance, limiting their market potential. Furthermore, the cost of developing a new antibiotic can be very high, often exceeding $1 billion [22].
-
Regulatory Challenges: The regulatory pathway for approving new antibiotics can be lengthy and complex. The FDA requires extensive clinical trials to demonstrate the safety and efficacy of new antibiotics, which can be time-consuming and expensive.
3.2. Strategies for Novel Antibiotic Development
Despite these challenges, there is renewed interest in developing new antibiotics. Several strategies are being pursued to overcome the obstacles to antibiotic innovation, including:
-
Target-Based Drug Discovery: This approach involves identifying essential bacterial targets and developing drugs that specifically inhibit these targets. Targets that are unique to bacteria or highly conserved across multiple species are particularly attractive [23].
-
Whole-Cell Screening: This approach involves screening large libraries of compounds for their ability to inhibit bacterial growth. This approach can identify compounds that act through novel mechanisms of action [24].
-
Structure-Based Drug Design: This approach involves using the three-dimensional structure of a bacterial target to design drugs that bind to and inhibit the target. This approach can be used to optimize the potency and selectivity of new antibiotics [25].
-
Fragment-Based Drug Discovery: This approach involves identifying small chemical fragments that bind to a bacterial target and then linking these fragments together to create a more potent drug [26].
-
Repurposing Existing Drugs: This approach involves identifying existing drugs that have antibacterial activity. This can be a faster and less expensive way to develop new antibiotics [27].
3.3. Emerging Antibiotic Classes and Approaches
-
Lipopeptides: These antibiotics, such as daptomycin, disrupt bacterial cell membrane function by inserting into the membrane and causing depolarization [28]. Daptomycin is effective against Gram-positive bacteria, including MRSA and VRE.
-
Oxazolidinones: These antibiotics, such as linezolid and tedizolid, inhibit protein synthesis by binding to the 23S rRNA component of the 50S ribosomal subunit. Oxazolidinones are effective against Gram-positive bacteria, including MRSA and VRE [29].
-
Glycylcyclines: These antibiotics, such as tigecycline, are tetracycline derivatives that have been modified to overcome tetracycline resistance mechanisms. Glycylcyclines bind to the 30S ribosomal subunit and inhibit protein synthesis [30].
-
Siderophore Conjugates: These antibiotics are conjugated to siderophores, molecules that bacteria use to acquire iron. The siderophore helps to transport the antibiotic into the bacterial cell, increasing its efficacy [31]. Cefiderocol is a siderophore cephalosporin that has shown activity against multidrug-resistant Gram-negative bacteria.
-
Boron-Containing Antibiotics: These antibiotics, such as vaborbactam, inhibit bacterial beta-lactamases, restoring the activity of beta-lactam antibiotics against resistant bacteria [32]. Vaborbactam is often used in combination with meropenem to treat carbapenem-resistant Enterobacteriaceae (CRE) infections.
-
Peptide Deformylase (PDF) Inhibitors: PDF is an essential bacterial enzyme involved in protein synthesis initiation. Inhibitors of PDF are being developed as potential broad-spectrum antibiotics [33].
-
CRISPR-Based Antimicrobials: The CRISPR-Cas system is a bacterial defense mechanism that can be used to target and kill bacteria. CRISPR-based antimicrobials are being developed to target specific bacterial genes, leading to cell death [34].
Many thanks to our sponsor Esdebe who helped us prepare this research report.
4. Antimicrobial Stewardship: Optimizing Antibiotic Use
Antimicrobial stewardship programs (ASPs) are essential for optimizing antibiotic use and preventing the further emergence of resistance. ASPs aim to improve patient outcomes by ensuring that antibiotics are used appropriately, only when necessary, and at the correct dose and duration [35].
4.1. Key Components of Antimicrobial Stewardship Programs
-
Formulary Restriction: Limiting the availability of certain antibiotics to restrict their use to specific indications [36].
-
Preauthorization: Requiring approval from an infectious disease specialist or other designated healthcare professional before certain antibiotics can be prescribed [37].
-
Prospective Audit and Feedback: Reviewing antibiotic prescriptions and providing feedback to prescribers on their appropriateness [38].
-
Guidelines and Education: Developing and implementing evidence-based guidelines for antibiotic use and providing education to healthcare professionals on antibiotic resistance and stewardship principles [39].
-
De-escalation of Therapy: Switching from broad-spectrum antibiotics to narrower-spectrum antibiotics once the causative organism and its susceptibility are known [40].
-
Dose Optimization: Ensuring that antibiotics are dosed appropriately to achieve optimal clinical outcomes while minimizing toxicity [41].
-
Duration Optimization: Using the shortest effective duration of antibiotic therapy to minimize the risk of resistance [42].
4.2. Impact of Antimicrobial Stewardship Programs
ASPs have been shown to be effective in reducing antibiotic use, decreasing the incidence of antibiotic-resistant infections, and improving patient outcomes [43]. Studies have demonstrated that ASPs can significantly reduce the use of broad-spectrum antibiotics, such as carbapenems and fluoroquinolones, and decrease the incidence of Clostridioides difficile infection [44]. ASPs can also improve the appropriateness of antibiotic prescribing, leading to better patient outcomes and reduced healthcare costs.
Many thanks to our sponsor Esdebe who helped us prepare this research report.
5. Alternative Therapies for Bacterial Infections
In addition to developing new antibiotics, alternative therapies are being explored as potential complements or replacements for traditional antibiotics. These therapies include:
5.1. Phage Therapy
Phage therapy involves using bacteriophages, viruses that infect and kill bacteria, to treat bacterial infections. Phages are highly specific for their target bacteria, minimizing the risk of disrupting the host microbiome. Phage therapy has shown promise in treating infections caused by multidrug-resistant bacteria [45]. However, challenges remain in developing phage therapies, including the need to identify and characterize phages that are effective against specific bacteria, the potential for bacteria to develop resistance to phages, and the regulatory hurdles to approving phage therapies [46].
5.2. Immunotherapy
Immunotherapy involves using the host’s immune system to fight bacterial infections. This can be achieved through various approaches, including:
-
Monoclonal Antibodies: Developing antibodies that target specific bacterial antigens, enhancing the host’s immune response [47].
-
Vaccines: Developing vaccines that stimulate the host’s immune system to produce antibodies against specific bacteria [48].
-
Cytokine Therapy: Using cytokines, signaling molecules that regulate the immune system, to enhance the host’s immune response to bacterial infections [49].
5.3. Other Alternative Therapies
-
Antimicrobial Peptides: These peptides are produced by many organisms as part of their innate immune system and have broad-spectrum antimicrobial activity [50].
-
Probiotics: These are live microorganisms that, when administered in adequate amounts, confer a health benefit on the host. Probiotics can help to prevent bacterial infections by competing with pathogenic bacteria and stimulating the host’s immune system [51].
-
Essential Oils: Some essential oils have antimicrobial activity and may be useful in treating bacterial infections [52].
Many thanks to our sponsor Esdebe who helped us prepare this research report.
6. Conclusion: Charting the Future of Antibiotic Research and Development
The antibiotic resistance crisis demands a multifaceted approach that encompasses the development of novel antibiotics, the implementation of robust antimicrobial stewardship programs, and the exploration of alternative therapies. Sustained investment in research and development is crucial for identifying new targets, developing innovative drugs, and overcoming the economic and regulatory hurdles that impede antibiotic innovation. Collaborative efforts involving academia, industry, government, and international organizations are essential for addressing this global health threat.
The future of antibiotic research and development will likely focus on several key areas:
-
Personalized Medicine: Tailoring antibiotic therapy to the individual patient based on their genetic makeup, immune status, and the specific characteristics of the infecting bacteria [53].
-
Diagnostics: Developing rapid and accurate diagnostic tests that can identify antibiotic-resistant bacteria and guide antibiotic prescribing [54].
-
Prevention: Implementing strategies to prevent bacterial infections, such as vaccination, improved hygiene, and infection control measures [55].
The challenge of antibiotic resistance is formidable, but with continued innovation, collaboration, and a commitment to responsible antibiotic use, we can safeguard the efficacy of antimicrobial therapies and protect public health.
Many thanks to our sponsor Esdebe who helped us prepare this research report.
References
[1] World Health Organization. (2014). Antimicrobial resistance: global report on surveillance 2014. World Health Organization.
[2] World Health Organization. (2023). Antimicrobial resistance. Retrieved from https://www.who.int/news-room/fact-sheets/detail/antimicrobial-resistance
[3] Gupta, K., Hooton, T. M., Naber, K. G., Wullt, B., Colgan, R., Miller, L. G., … & Stamm, W. E. (2011). International clinical practice guidelines for the treatment of acute uncomplicated cystitis and pyelonephritis in women: A 2010 update by the Infectious Diseases Society of America and the European Society for Microbiology and Infectious Diseases. Clinical Infectious Diseases, 52(5), e103-e120.
[4] Waxman, D. J., & Strominger, J. L. (1983). Penicillin-binding proteins and the mechanism of action of beta-lactam antibiotics. Annual Review of Biochemistry, 52(1), 825-869.
[5] van Hal, S. J., Paterson, D. L., & Lodise, T. P. (2013). Systematic review and meta-analysis of the comparative efficacy of vancomycin and teicoplanin for treatment of staphylococcus aureus infections. The American Journal of Medicine, 126(9), 750-760.e11.
[6] Krause, K. M., Serio, A. W., Spellberg, B., & Abrantes, J. A. (2016). Aminoglycosides: an overview. Cold Spring Harbor Perspectives in Medicine, 6(6), a027029.
[7] Chopra, I., & Roberts, M. C. (2001). Tetracycline antibiotics: mode of action, applications, molecular biology, and epidemiology of resistance. Microbiology and Molecular Biology Reviews, 65(2), 232-260.
[8] Weisblum, B. (1995). Erythromycin resistance. Antimicrobial Agents and Chemotherapy, 39(3), 577-585.
[9] Drlica, K., & Zhao, X. (1997). DNA gyrase, topoisomerase IV, and the 4-quinolones. Microbiology and Molecular Biology Reviews, 61(3), 377-392.
[10] Campbell, E. A., Korzheva, N., Mustaev, A., Murakami, K. S., Nair, S., Goldfarb, A., & Darst, S. A. (2001). The structure of Escherichia coli RNA polymerase holoenzyme at 2.8 Å resolution. Cell, 104(6), 901-912.
[11] Sköld, O. (2001). Sulfonamides and trimethoprim: mechanism of action and resistance. Reviews of Infectious Diseases, 4(Suppl 4), S321-S324.
[12] Bush, K. (2018). Beta-lactamase nomenclature and classification: an update. Clinical Microbiology Reviews, 31(4), e00047-18.
[13] Ramirez, M. S., & Tolmasky, M. E. (2010). Aminoglycoside modifying enzymes. Drug Resistance Updates, 13(6), 151-171.
[14] Fishovitz, J., & Hermoso, J. A. (2015). Penicillin-binding proteins and beta-lactam resistance: structure and mechanism. Antimicrobial Agents and Chemotherapy, 59(11), 6435-6446.
[15] Vester, B., & Douthwaite, S. (2001). Macrolide resistance conferred by modification of 23S rRNA. Antimicrobial Agents and Chemotherapy, 45(1), 1-12.
[16] Hooper, D. C., & Jacoby, G. A. (2016). Mechanisms of quinolone resistance. Cold Spring Harbor Perspectives in Medicine, 6(10), a025635.
[17] Blair, J. M. A., Webber, M. A., Baylay, A. J., Ogbolu, D. O., Piddock, L. J. V. (2015). Molecular mechanisms of antibiotic resistance. Nature Reviews Microbiology, 13(1), 42-51.
[18] Nikaido, H. (2009). Permeability of outer membranes of gram-negative bacteria. Biochimica et Biophysica Acta (BBA)-Biomembranes, 1798(11), 2237-2247.
[19] Flensburg, J., Dahlberg, C., Cardell, L. O., & Hughes, D. (2009). Selection for trimethoprim resistance promotes high-level chromosomal mutations in Escherichia coli. Genetics, 182(3), 739-748.
[20] Høiby, N., Bjarnsholt, T., Moser, C., Bassi, G. L., Coenye, T., Donelli, G., … & Givskov, M. (2015). ESCMID guideline for the diagnosis and treatment of biofilm infections. Clinical Microbiology and Infection, 21(Suppl 1), S1-S25.
[21] Spellberg, B., Guidos, R. J., Gilbert, D., Bradley, J. S., Boucher, H. W., Link, A. S., … & Powers, J. H. (2008). The epidemic of antibiotic-resistant infections: a call to action for the medical community. Archives of Internal Medicine, 168(13), 1357-1362.
[22] Outterson, K., Powers, J. H., & Patel, B. N. (2015). Assessment of clinical development programs for new antibacterial drugs. Clinical Infectious Diseases, 61(suppl 1), S36-S42.
[23] Payne, D. J., Gwynn, M. N., Holmes, D. J., & Pompliano, D. L. (2007). Drugs for bad bugs: confronting the challenges of antibacterial discovery. Nature Reviews Drug Discovery, 6(1), 29-40.
[24] Silver, L. L. (2011). Challenges of antibacterial discovery. Trends in Pharmacological Sciences, 32(2), 72-78.
[25] Blundell, T. L., Jhoti, H., & Abell, C. (2002). High-throughput X-ray crystallography for drug discovery. Nature Reviews Drug Discovery, 1(1), 45-54.
[26] Erlanson, D. A., Fesik, S. W., Hubbard, R. E., Jahnke, W., & Shuker, S. B. (2016). Twenty years on: the impact of fragment-based drug discovery. Nature Reviews Drug Discovery, 15(9), 605-619.
[27] Chong, C. R., & Sullivan Jr, D. J. (2007). New uses for old drugs. Nature, 448(7154), 645-646.
[28] Steenbergen, J. N., Alder, J., Thorne, G. M., & Tally, F. P. (2005). Daptomycin: mechanism of action, resistance, and spectrum of activity. Journal of Antimicrobial Chemotherapy, 55(3), 283-288.
[29] Livermore, D. M. (2011). Tigecycline: what is its place in therapy?. International Journal of Antimicrobial Agents, 37(6), 501-513.
[30] Zhanel, G. G., Cheung, D., Adam, H., Zelenitsky, S., Golden, A. R., Schweizer, F., … & Walkty, A. J. (2010). Review of tigecycline, a novel glycylcycline antimicrobial agent. Expert Opinion on Pharmacotherapy, 11(13), 2191-2222.
[31] Page, M. G. P., Dantier, C., Desarbre, E., Hernandez, D., Lawton, E. M., Lemaire, S., … & Watkins, W. J. (2018). Cefiderocol, a novel siderophore cephalosporin: in vitro activity against Gram-negative bacteria. Antimicrobial Agents and Chemotherapy, 62(11), e01314-18.
[32] Lomovskaya, O., Sun, D., Senderovich, Y., Lee, A., Nelson, K., Tsivkovski, R., … & Dudley, M. N. (2017). Vaborbactam: spectrum of in vitro activity and impact on meropenem activity against carbapenem-resistant Enterobacteriaceae. Antimicrobial Agents and Chemotherapy, 61(11), e01427-17.
[33] Becker, A., Schmeisser, C., & Bott, M. (2013). Peptide deformylase as a target for antibacterial drug development. Anti-Infective Agents in Medicinal Chemistry, 11(4), 305-315.
[34] Bikard, D., Euler, C. W., Jiang, W., Nussenzweig, D. R., Goldberg, G. W., Duportet, X., … & Marraffini, L. A. (2014). Exploiting CRISPR-Cas immunity to develop sequence-specific antimicrobials. Nature Biotechnology, 32(11), 1146-1148.
[35] Barlam, T. F., Cosgrove, S. E., Abbo, L. M., MacDougall, C., Schuetz, A. N., Septimus, E. J., … & Wright, M. O. (2016). Implementing an antibiotic stewardship program: guidelines by the Infectious Diseases Society of America and the Society for Healthcare Epidemiology of America. Clinical Infectious Diseases, 62(10), e51-e77.
[36] Goff, D. A., Kullar, R., Goldstein, E. J., Gilchrist, M., Zilberberg, M. D., & Nathwani, D. (2017). Antimicrobial stewardship: how the microbiology laboratory can right the ship. Clinical Microbiology Reviews, 30(2), 381-402.
[37] Davey, P., Brown, E., Fenelon, L., Finch, R., Gould, I., Howard, P., … & Wilcox, M. (2005). Interventions to improve antibiotic prescribing practices for hospital inpatients. Cochrane Database of Systematic Reviews, (4).
[38] Goff, D. A., File, T. M., Jr, & Bradley, J. S. (2012). Practical implementation of antibiotic stewardship programs: consensus from the Society for Healthcare Epidemiology of America. Infection Control & Hospital Epidemiology, 33(4), 323-328.
[39] Dellit, T. H., Owens, R. C., McGowan Jr, J. E., Gerding, D. N., Weinstein, R. A., Burke, J. P., … & Fishman, N. O. (2007). Infectious Diseases Society of America and the Society for Healthcare Epidemiology of America guidelines for developing an institutional program to enhance antimicrobial stewardship. Clinical Infectious Diseases, 44(2), 159-177.
[40] Hecker, M. T., Donskey, C. J., London, M. L., & Jump, R. L. (2006). Effect of antibiotic stewardship on the incidence of infection with antibiotic-resistant bacteria and Clostridium difficile infection in a long-term care facility. Infection Control & Hospital Epidemiology, 27(1), 1-7.
[41] Roberts, J. A., Paul, S. K., Akova, M., Bassetti, M., De Waele, J. J., Dimopoulos, G., … & Lipman, J. (2014). DALI: defining antibiotic levels in intensive care unit patients: are current beta-lactam antibiotic doses sufficient for critically ill patients?. Clinical Infectious Diseases, 58(8), 1072-1083.
[42] Spellberg, B., Rice, L. B., & Nouri, S. (2014). Shorter is better: antimicrobial stewardship considerations for duration of therapy. Clinical Infectious Diseases, 59(Suppl 3), S151-S157.
[43] Nathwani, D., Varghese, D., Stephens, J. W., Ramsbottom, T., Findlay, D., & Oliver, I. (2019). Value of hospital antimicrobial stewardship programmes [comment]. The Lancet, 393(10175), 981-990.
[44] Fridkin, S. K., Baggs, J., Fagan, R., Magill, S. S., Pollack, L. A., Weisenberg, S., … & Jernigan, J. A. (2014). Vital signs: improving antibiotic use among hospitalized patients. Morbidity and Mortality Weekly Report, 63(9), 194-200.
[45] Dedrick, R. M., Guerrero-Bustamante, C. A., Garlena, R. A., Russell, D. A., Ford, K., Harris, K., … & Spencer, H. (2019). Phage therapy of Mycobacterium abscessus infection. Nature Medicine, 25(5), 730-733.
[46] Gill, J. J., & Hyman, P. (2010). Phage therapy: past, present and future. Future Microbiology, 5(4), 565-576.
[47] Casadevall, A., & Dadachova, E. (2014). Antibody-based therapies for infectious diseases. Nature Reviews Microbiology, 12(2), 123-134.
[48] Plotkin, S. A., Orenstein, W. A., & Offit, P. A. (2017). Vaccines (7th ed.). Elsevier.
[49] Romagnani, S. (1999). Th1/Th2 cells. Cytokine-induced developmental pathways in human lymphocytes. Springer, Berlin, Heidelberg.
[50] Hancock, R. E., & Sahl, H. G. (2006). Antimicrobial and host-defense peptides as new anti-infective therapeutic strategies. Nature Biotechnology, 24(12), 1551-1557.
[51] Guarner, F., Sanders, M. E., Eliakim, R., Fedorak, R., Gangl, A., Garisch, J., … & Gibson, G. R. (2005). Probiotic and prebiotic claims in functional foods: a consensus report. International Journal of Food Microbiology, 101(3), 251-275.
[52] Reichling, J., Schnitzler, P., Suschke, U., & Saller, R. (2009). Essential oils of aromatic plants with antibacterial, antifungal, antiviral, and cytotoxic properties–an overview. Complementary Medicine Research, 16(2), 79-90.
[53] Ashley, E. A. (2015). Towards precision medicine. The Lancet, 386(9995), 905-906.
[54] O’Neill, J. (2016). Tackling drug-resistant infections globally: final report and recommendations. Review on Antimicrobial Resistance.
[55] Dancer, S. J. (2008). Controlling hospital-acquired infection: focus on the role of the environment and new technologies. Clinical Microbiology Reviews, 21*(3), 584-616.
Alternative therapies, like phage therapy, sound straight out of a sci-fi film! Imagine releasing tiny virus assassins to take down superbugs. I wonder if they need a tiny cloak and dagger… or maybe just a good targeting system? Let’s hope the future of fighting infections is this cool!
That’s a great point about the targeting system! Precision is key for phage therapy. Researchers are actively working on engineering phages with enhanced specificity to target resistant bacteria, minimizing impact on the beneficial microbiome. It’s a fascinating area of development!
Editor: MedTechNews.Uk
Thank you to our Sponsor Esdebe
This report highlights the urgent need for innovative drug delivery systems. Has there been any progress in developing targeted nanoparticles or other advanced methods to enhance antibiotic penetration into biofilms, addressing a significant hurdle in treating persistent infections?