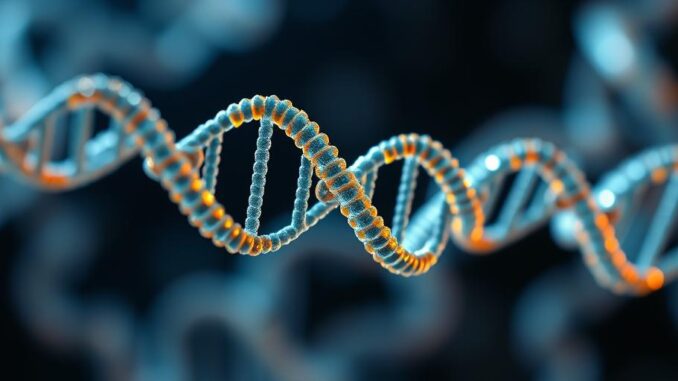
Abstract
Genome editing technologies, particularly CRISPR-Cas9, have revolutionized biological research and hold immense promise for treating genetic diseases. This report provides a comprehensive overview of the field, extending beyond the immediate therapeutic applications to examine the underlying scientific principles, technological advancements, ethical considerations, regulatory landscape, and the broader societal impact of genome editing. We delve into the mechanisms of various editing systems, including base editing and prime editing, and discuss their advantages and limitations. Furthermore, we critically assess the challenges associated with off-target effects, delivery strategies, and immune responses. The ethical and societal implications of germline editing, enhancement applications, and equitable access to these technologies are explored in detail. Finally, we examine the current regulatory frameworks and discuss the need for adaptive and globally harmonized policies to ensure the responsible development and deployment of genome editing technologies.
Many thanks to our sponsor Esdebe who helped us prepare this research report.
1. Introduction
Genome editing, the ability to precisely alter the DNA sequence of living organisms, has rapidly transformed biological research and holds unprecedented potential for treating a wide range of diseases. While gene therapy approaches involving the insertion of corrected gene copies have been under development for decades, the advent of programmable nucleases such as zinc-finger nucleases (ZFNs), transcription activator-like effector nucleases (TALENs), and, most notably, the clustered regularly interspaced short palindromic repeats (CRISPR)-CRISPR-associated protein 9 (Cas9) system, has dramatically increased the efficiency, precision, and accessibility of genome editing. CRISPR-Cas9, derived from the adaptive immune system of bacteria, has become the dominant tool due to its simplicity, versatility, and cost-effectiveness (Doudna & Charpentier, 2014).
This report aims to provide a comprehensive overview of the field of genome editing, going beyond the immediate focus on therapeutic applications. We will examine the fundamental principles of various editing technologies, their current state of development, the associated ethical and societal concerns, and the evolving regulatory landscape. Specifically, we will address the following key areas:
- Mechanisms of Genome Editing Technologies: A detailed examination of CRISPR-Cas9, base editing, prime editing, and other emerging genome editing tools.
- Challenges and Limitations: Critical assessment of off-target effects, delivery strategies, immune responses, and other technical hurdles.
- Therapeutic Applications: Overview of current clinical trials and preclinical research targeting various genetic diseases and acquired conditions.
- Ethical Considerations: In-depth discussion of the ethical implications of germline editing, enhancement applications, and equitable access to genome editing technologies.
- Regulatory Landscape: Analysis of the current regulatory frameworks and the need for adaptive and globally harmonized policies.
- Societal Impact: Exploration of the broader societal implications of genome editing, including public perception, risk communication, and potential for misuse.
By addressing these areas, this report seeks to provide a nuanced and comprehensive perspective on the evolving landscape of genome editing, informing researchers, policymakers, and the public about the opportunities and challenges associated with this transformative technology.
Many thanks to our sponsor Esdebe who helped us prepare this research report.
2. Mechanisms of Genome Editing Technologies
The power of genome editing lies in its ability to precisely target and modify specific DNA sequences within a cell or organism. Several technologies have been developed to achieve this goal, each with its own advantages and limitations. We will now delve into the mechanisms of CRISPR-Cas9, base editing, and prime editing, representing the most prominent and rapidly evolving tools in the field.
2.1 CRISPR-Cas9: The Revolution in Genome Editing
The CRISPR-Cas9 system, derived from the adaptive immune system of bacteria, is a powerful and versatile genome editing tool. It relies on two key components: the Cas9 protein, an RNA-guided endonuclease, and a guide RNA (gRNA), a short RNA molecule that directs Cas9 to the target DNA sequence (Jinek et al., 2012). The gRNA consists of two regions: a CRISPR RNA (crRNA) sequence that is complementary to the target DNA and a trans-activating crRNA (tracrRNA) sequence that binds to Cas9. The gRNA directs Cas9 to the target DNA sequence through base pairing, typically requiring a protospacer adjacent motif (PAM) sequence downstream of the target site. Upon binding to the target DNA, Cas9 introduces a double-strand break (DSB). This DSB can be repaired by one of two major pathways: non-homologous end joining (NHEJ) or homology-directed repair (HDR).
- NHEJ: This pathway is error-prone and often leads to the insertion or deletion of nucleotides (indels) at the break site, resulting in gene disruption. NHEJ is the dominant repair pathway in most cell types and is often used to knockout gene function.
- HDR: This pathway uses a provided DNA template to repair the DSB, allowing for precise gene editing. HDR is less efficient than NHEJ but enables the insertion of new DNA sequences or the correction of existing mutations. The efficiency of HDR can be improved by optimizing the DNA template and using small molecule inhibitors of the NHEJ pathway.
The simplicity and versatility of CRISPR-Cas9 have made it the dominant tool in genome editing research. However, it is important to note that the system is not without its limitations. Off-target effects, where Cas9 cuts at unintended sites in the genome, are a major concern. The efficiency of HDR is also relatively low in many cell types, limiting its applicability for precise gene editing.
2.2 Base Editing: Precise Single-Base Conversions
Base editing offers a more precise approach to genome editing by enabling the direct conversion of one DNA base into another, without introducing DSBs. Base editors consist of a catalytically dead Cas9 (dCas9) or a Cas9 nickase (nCas9) fused to a deaminase enzyme. There are two main types of base editors: cytosine base editors (CBEs) and adenine base editors (ABEs).
- CBEs: These editors convert cytosine (C) to thymine (T) by deaminating cytosine to uracil (U), which is then recognized as thymine during DNA replication. CBEs typically use an APOBEC family deaminase enzyme, such as APOBEC1. CBEs are effective at correcting point mutations that involve C to T or G to A transitions.
- ABEs: These editors convert adenine (A) to guanine (G) by deaminating adenine to inosine (I), which is then recognized as guanine during DNA replication. ABEs use a modified tRNA adenosine deaminase enzyme. ABEs are effective at correcting point mutations that involve A to G or T to C transitions.
Base editing offers several advantages over CRISPR-Cas9. By avoiding DSBs, base editing reduces the risk of off-target effects and unwanted indels. Base editing also has the potential to be more efficient than HDR-based gene editing in certain contexts. However, base editing is limited to single-base conversions and cannot be used to insert or delete large DNA sequences. Furthermore, off-target deamination of RNA and DNA remains a concern and ongoing research is focused on improving the specificity and efficiency of base editors (Komor et al., 2016).
2.3 Prime Editing: A Versatile and Precise Genome Editing Tool
Prime editing is a more recent development in genome editing that offers greater versatility and precision compared to CRISPR-Cas9 and base editing. Prime editing uses a Cas9 nickase (nCas9) fused to a reverse transcriptase enzyme, guided by a prime editing guide RNA (pegRNA). The pegRNA contains both a target-binding sequence and a reverse transcriptase template sequence encoding the desired edit (Anzalone et al., 2019).
During prime editing, the nCas9 nicks the target DNA strand, and the reverse transcriptase uses the pegRNA template to synthesize a new DNA strand containing the desired edit. This new DNA strand then displaces the original DNA strand, and the cell’s DNA repair machinery resolves the mismatch, resulting in the incorporation of the desired edit into the genome.
Prime editing offers several advantages over other genome editing technologies:
- Versatility: Prime editing can be used to perform a wide range of edits, including single-base substitutions, insertions, and deletions.
- Precision: Prime editing is highly precise and minimizes the risk of off-target effects and unwanted indels.
- Reduced Dependence on HDR: Prime editing does not rely on HDR, which is often inefficient in many cell types.
While prime editing is a promising technology, it is still relatively new and faces several challenges. The efficiency of prime editing can be lower than that of CRISPR-Cas9 or base editing in some contexts. Further optimization of the pegRNA design and delivery strategies is needed to improve the efficiency and applicability of prime editing. The size of the editing payload is also restricted.
2.4 Other Genome Editing Technologies
Beyond CRISPR-Cas9, base editing, and prime editing, several other genome editing technologies are under development. These include:
- Zinc-Finger Nucleases (ZFNs): ZFNs are engineered proteins that consist of a zinc-finger DNA-binding domain fused to a FokI nuclease domain. ZFNs can be designed to target specific DNA sequences and introduce DSBs. While ZFNs were among the first programmable nucleases developed, they are more complex to design and synthesize than CRISPR-Cas9.
- Transcription Activator-Like Effector Nucleases (TALENs): TALENs are similar to ZFNs but use a different DNA-binding domain based on transcription activator-like effectors (TALEs) from Xanthomonas bacteria. TALENs are also more complex to design and synthesize than CRISPR-Cas9.
- CRISPR-Cas12a (Cpf1): Cas12a is another type of CRISPR-associated protein that differs from Cas9 in several ways. Cas12a recognizes a different PAM sequence than Cas9 and generates a staggered DSB. Cas12a has been shown to have higher specificity than Cas9 in some contexts.
The choice of genome editing technology depends on the specific application and the desired outcome. CRISPR-Cas9 remains the most widely used tool due to its simplicity and versatility. Base editing and prime editing offer more precise and versatile approaches for specific types of edits. ZFNs and TALENs are less commonly used due to their complexity. Further research and development are needed to improve the efficiency, specificity, and applicability of all genome editing technologies.
Many thanks to our sponsor Esdebe who helped us prepare this research report.
3. Challenges and Limitations
Despite the remarkable progress in genome editing, several challenges and limitations remain that need to be addressed to fully realize the therapeutic potential of these technologies. These challenges include off-target effects, delivery strategies, immune responses, and mosaicism.
3.1 Off-Target Effects: A Major Safety Concern
Off-target effects, where the genome editing tool cuts or modifies DNA at unintended sites in the genome, are a major safety concern. Off-target effects can lead to unintended mutations, chromosomal rearrangements, and potentially even cancer. The risk of off-target effects is particularly high with CRISPR-Cas9, where the gRNA can bind to DNA sequences that are similar to the target site. Several strategies have been developed to minimize off-target effects, including:
- Improved gRNA Design: Algorithms and software tools have been developed to design gRNAs with higher specificity and lower off-target potential.
- High-Fidelity Cas9 Variants: Engineered Cas9 variants with increased specificity and reduced off-target activity have been developed.
- Paired Nickases: Using two Cas9 nickases to target the same site can reduce off-target effects by requiring two independent binding events for a DSB to occur.
- Off-Target Detection Methods: Various methods have been developed to detect and quantify off-target effects, including GUIDE-seq, CIRCLE-seq, and Digenome-seq. These methods can help identify potential off-target sites and optimize the editing strategy.
While these strategies have significantly reduced the risk of off-target effects, it is important to note that no genome editing tool is completely free of off-target activity. Careful design, rigorous testing, and thorough characterization of off-target effects are essential for ensuring the safety of genome editing therapies.
3.2 Delivery Strategies: Getting the Editing Tool to the Right Place
Efficient and targeted delivery of the genome editing tool to the desired cells or tissues is another major challenge. The delivery method must be safe, effective, and specific to the target cells. Several delivery methods are being explored, including:
- Viral Vectors: Adeno-associated viruses (AAVs), lentiviruses, and adenoviruses are commonly used to deliver genome editing tools. Viral vectors can be highly efficient at transducing cells, but they can also elicit immune responses and have limited cargo capacity.
- Non-Viral Vectors: Lipid nanoparticles (LNPs), exosomes, and electroporation are non-viral delivery methods that offer several advantages over viral vectors, including lower immunogenicity and larger cargo capacity. However, non-viral vectors are generally less efficient at transducing cells than viral vectors.
- Direct Delivery: In some cases, the genome editing tool can be directly delivered to the target tissue via injection or other means. This approach is limited to tissues that are easily accessible.
The choice of delivery method depends on the specific application and the target tissue. For example, AAV vectors are commonly used to deliver genome editing tools to the liver, while LNPs are being used to deliver mRNA encoding Cas9 to various tissues. Further research is needed to develop more efficient, targeted, and safe delivery methods for genome editing tools.
3.3 Immune Responses: A Potential Barrier to Therapeutic Efficacy
The immune system can recognize and attack genome editing tools, particularly viral vectors and Cas9 protein. Immune responses can reduce the efficacy of genome editing therapies and potentially cause adverse effects. Several strategies are being explored to minimize immune responses, including:
- Immunosuppression: Using immunosuppressant drugs to suppress the immune system.
- Engineering Cas9 Variants with Reduced Immunogenicity: Modifying the Cas9 protein to reduce its immunogenicity.
- Using Autologous Cells: Editing cells outside the body (ex vivo) and then transplanting them back into the patient can reduce the risk of immune responses.
- Local Delivery: Delivering the genome editing tool directly to the target tissue can minimize systemic exposure and reduce the risk of immune responses.
Understanding and managing immune responses are crucial for the successful development of genome editing therapies.
3.4 Mosaicism: Incomplete Editing in Target Cells
Mosaicism refers to the presence of both edited and unedited cells in the target tissue or organism. Mosaicism can result in incomplete therapeutic efficacy, particularly if a high percentage of cells remain unedited. Several factors can contribute to mosaicism, including inefficient delivery, variable editing efficiency, and cell-to-cell variability. Strategies to minimize mosaicism include:
- Optimizing Delivery and Editing Efficiency: Improving the efficiency of delivery and editing can increase the percentage of edited cells.
- Selecting for Edited Cells: Using selection markers to enrich for edited cells.
- Multiple Editing Rounds: Performing multiple rounds of editing to increase the percentage of edited cells.
Minimizing mosaicism is important for achieving optimal therapeutic outcomes.
Many thanks to our sponsor Esdebe who helped us prepare this research report.
4. Therapeutic Applications
Genome editing technologies have the potential to revolutionize the treatment of a wide range of diseases, including genetic disorders, infectious diseases, and cancer. Numerous clinical trials and preclinical research efforts are underway to explore the therapeutic applications of genome editing. We will now discuss some of the most promising therapeutic applications.
4.1 Genetic Disorders
Genetic disorders, caused by mutations in genes, are a major target for genome editing therapies. Genome editing can be used to correct the mutated gene, disrupt the expression of the mutated gene, or insert a corrected copy of the gene. Several genetic disorders are currently being targeted by genome editing therapies, including:
- Cystic Fibrosis: CRISPR-Cas9 is being used to correct the most common mutation in the CFTR gene, which causes cystic fibrosis.
- Sickle Cell Disease: CRISPR-Cas9 is being used to edit the BCL11A gene in hematopoietic stem cells, leading to increased production of fetal hemoglobin and reduced sickling.
- Beta-Thalassemia: Similar to sickle cell disease, CRISPR-Cas9 is being used to edit the BCL11A gene in hematopoietic stem cells to increase fetal hemoglobin production.
- Duchenne Muscular Dystrophy: CRISPR-Cas9 is being used to delete exons in the dystrophin gene, restoring the reading frame and producing a truncated but functional protein.
- Huntington’s Disease: CRISPR-Cas9 is being used to disrupt the expression of the mutated huntingtin gene, which causes Huntington’s disease.
- Spinal Muscular Atrophy (SMA): While not directly editing the mutated gene, genome editing strategies are being explored to enhance the expression of the backup SMN2 gene, compensating for the loss of function of SMN1.
4.2 Infectious Diseases
Genome editing can also be used to treat infectious diseases by targeting the pathogen’s genome or by enhancing the host’s immune response. For example:
- HIV: CRISPR-Cas9 is being used to disrupt the HIV genome in infected cells, preventing viral replication. CRISPR-Cas9 is also being used to edit the CCR5 gene in immune cells, making them resistant to HIV infection.
- Hepatitis B Virus (HBV): CRISPR-Cas9 is being used to disrupt the HBV genome in infected liver cells, preventing viral replication.
- COVID-19: CRISPR-based diagnostics are being used to detect the SARS-CoV-2 virus. In addition, CRISPR-Cas9 is being explored as a potential therapeutic to target and destroy the viral RNA.
4.3 Cancer
Genome editing is being explored as a potential therapy for cancer by targeting cancer-causing genes, enhancing the immune response against cancer cells, or delivering therapeutic genes to cancer cells. For example:
- Targeting Cancer-Causing Genes: CRISPR-Cas9 is being used to disrupt the expression of oncogenes or to correct mutations in tumor suppressor genes.
- Enhancing the Immune Response: CRISPR-Cas9 is being used to engineer immune cells to target and kill cancer cells. This approach, known as CAR-T cell therapy, has shown promising results in treating certain types of leukemia and lymphoma.
- Delivering Therapeutic Genes: CRISPR-Cas9 is being used to deliver therapeutic genes to cancer cells, such as genes that encode for cytotoxic proteins or immune-stimulating factors.
4.4 Ex Vivo vs. In Vivo Gene Editing
Genome editing therapies can be delivered either ex vivo or in vivo.
- Ex Vivo: In ex vivo gene editing, cells are extracted from the patient, edited in the laboratory, and then transplanted back into the patient. Ex vivo gene editing is typically used for blood disorders and immune disorders.
- In Vivo: In in vivo gene editing, the genome editing tool is delivered directly to the patient’s body, targeting the affected cells or tissues. In vivo gene editing is being explored for a wider range of diseases, including liver disorders, muscle disorders, and eye disorders.
The choice between ex vivo and in vivo gene editing depends on the specific disease and the target tissue. Ex vivo gene editing offers greater control over the editing process and reduces the risk of off-target effects, but it is more complex and time-consuming. In vivo gene editing is simpler and less time-consuming, but it is more challenging to control and carries a higher risk of off-target effects.
Many thanks to our sponsor Esdebe who helped us prepare this research report.
5. Ethical Considerations
The development and application of genome editing technologies raise profound ethical considerations that must be carefully addressed. These considerations include the ethics of germline editing, enhancement applications, and equitable access to genome editing technologies.
5.1 Germline Editing: A Contentious Issue
Germline editing, which involves modifying the DNA of sperm, eggs, or early embryos, is a particularly contentious issue. Germline edits are heritable, meaning that they will be passed down to future generations. Proponents of germline editing argue that it could be used to prevent the transmission of devastating genetic diseases. Opponents argue that it is unethical to make changes to the human germline that could have unintended consequences for future generations. The long-term effects of germline editing are unknown, and there is a risk that it could lead to unintended health problems or even alter the course of human evolution. Furthermore, the potential for unintended consequences in future generations weighs heavily against the potential benefits. The scientific community is largely in agreement that germline editing should not be pursued at this time, except in very limited circumstances and with strict oversight (Baltimore et al., 2015).
5.2 Enhancement Applications: Beyond Disease Treatment
Genome editing could potentially be used for enhancement purposes, such as increasing muscle mass, enhancing cognitive abilities, or altering physical appearance. The use of genome editing for enhancement raises ethical concerns about fairness, social justice, and the potential for creating a genetically engineered elite. There is a risk that enhancement applications could exacerbate existing social inequalities and lead to discrimination against individuals who have not been genetically enhanced. The distinction between therapy and enhancement is often blurry, and it is important to have a public discussion about the appropriate boundaries of genome editing technology.
5.3 Equitable Access: Ensuring Fair Distribution of Benefits
Genome editing therapies are likely to be expensive, raising concerns about equitable access. It is important to ensure that these therapies are available to all who need them, regardless of their socioeconomic status or geographic location. The development of affordable genome editing technologies and the implementation of fair and equitable healthcare policies are essential for ensuring equitable access. The ethical implications of withholding potentially life-saving treatments based on financial or social factors are significant and must be addressed proactively.
5.4 Informed Consent and Public Engagement
Informed consent is crucial for any medical intervention, including genome editing. Patients must be fully informed about the risks and benefits of genome editing therapies before making a decision. Public engagement is also essential for building trust and ensuring that genome editing technologies are used responsibly. Open and transparent discussions about the ethical implications of genome editing are needed to foster public understanding and support for these technologies.
Many thanks to our sponsor Esdebe who helped us prepare this research report.
6. Regulatory Landscape
The regulatory landscape for genome editing is complex and evolving. Different countries have different regulations regarding the use of genome editing technologies. The U.S. Food and Drug Administration (FDA) regulates genome editing therapies as drugs or biologics. In Europe, genome editing is regulated under the European Union’s (EU) framework for genetically modified organisms (GMOs). The EU’s regulations are generally more restrictive than those in the United States.
6.1 Harmonization of Regulations
There is a need for greater harmonization of regulations across countries to facilitate the development and deployment of genome editing technologies. Globally harmonized regulations would promote innovation, reduce regulatory uncertainty, and ensure that genome editing therapies are safe and effective. However, achieving global harmonization will be challenging due to differing ethical and societal values.
6.2 Adaptive Regulation
The regulatory landscape for genome editing must be adaptive to keep pace with the rapid advancements in the field. Regulations should be flexible enough to accommodate new technologies and applications while ensuring patient safety and ethical considerations. Adaptive regulation requires ongoing monitoring of scientific advancements, engagement with stakeholders, and a willingness to adjust regulations as needed. This also means that regulations must have clear criteria to distinguish between the different potential applications of the technology.
6.3 Public Trust and Transparency
Building public trust and ensuring transparency are essential for the responsible development and deployment of genome editing technologies. Regulatory agencies should be transparent in their decision-making processes and engage with the public to address concerns and build trust. Transparency in research and clinical trials is also crucial for fostering public confidence in genome editing technologies.
Many thanks to our sponsor Esdebe who helped us prepare this research report.
7. Societal Impact
Genome editing has the potential to have a profound impact on society, both positive and negative. It is important to consider the broader societal implications of genome editing and to engage in a public dialogue about the future of this technology.
7.1 Impact on Healthcare
Genome editing has the potential to revolutionize healthcare by providing new treatments for genetic diseases, infectious diseases, and cancer. Genome editing therapies could potentially cure diseases that are currently untreatable or poorly managed. This could lead to improved quality of life, increased lifespan, and reduced healthcare costs.
7.2 Impact on Agriculture
Genome editing can also be used to improve crops and livestock. Genome editing can be used to increase crop yields, enhance nutritional content, and improve resistance to pests and diseases. This could lead to increased food security and reduced reliance on pesticides and herbicides.
7.3 Economic Impact
The development and commercialization of genome editing technologies could have a significant economic impact. Genome editing companies are attracting significant investment, and the genome editing market is expected to grow rapidly in the coming years. Genome editing could also create new jobs in research, development, manufacturing, and healthcare.
7.4 Potential for Misuse
Genome editing technologies could potentially be misused for malicious purposes, such as creating bioweapons or genetically engineering humans for unethical purposes. It is important to be aware of the potential for misuse and to take steps to prevent it. International cooperation and responsible oversight are essential for preventing the misuse of genome editing technologies.
Many thanks to our sponsor Esdebe who helped us prepare this research report.
8. Conclusion
Genome editing technologies, particularly CRISPR-Cas9, have revolutionized biological research and hold immense promise for treating a wide range of diseases. While the field has made remarkable progress, several challenges and limitations remain, including off-target effects, delivery strategies, immune responses, and ethical considerations. Addressing these challenges and fostering public dialogue are essential for the responsible development and deployment of genome editing technologies.
The ethical implications of germline editing, enhancement applications, and equitable access must be carefully considered. Adaptive and globally harmonized regulations are needed to promote innovation, reduce regulatory uncertainty, and ensure patient safety. Transparency, public engagement, and international cooperation are crucial for building trust and preventing the misuse of genome editing technologies.
As genome editing technologies continue to evolve, it is essential to maintain a focus on both the potential benefits and the potential risks. By carefully considering the ethical, societal, and regulatory implications of genome editing, we can ensure that these powerful technologies are used responsibly to improve human health and well-being. In order to realize the full potential of genome editing, we must also promote interdisciplinary collaboration, fostering communication between scientists, ethicists, policymakers, and the public.
Many thanks to our sponsor Esdebe who helped us prepare this research report.
References
- Anzalone, A. V., Randolph, P. B., Davis, J. R., Sousa, A. A., Koblan, L. W., Levy, J. M., … & Liu, D. R. (2019). Search-and-replace genome editing without double-strand breaks. Nature, 576(7785), 149-157.
- Baltimore, D., Berg, P., Botchan, M., Carroll, D., Charo, R. A., Church, G., … & Zambidis, E. (2015). A prudent path forward for genomic engineering and germline gene modification. Science, 348(6230), 36-38.
- Doudna, J. A., & Charpentier, E. (2014). Genome editing. Science, 346(6213), 1258096.
- Jinek, M., Chylinski, K., Fonfara, I., Hauer, M., Doudna, J. A., & Charpentier, E. (2012). A programmable dual-RNA-guided DNA endonuclease in adaptive bacterial immunity. Science, 337(6096), 816-821.
- Komor, A. C., Kim, Y. B., Packer, M. S., Zuris, J. A., & Liu, D. R. (2016). Programmable editing of a target base in genomic DNA without double-stranded DNA cleavage. Nature, 533(7603), 420-424.
Germline editing, eh? So, we’re talking about potentially rewriting the human story, one embryo at a time? Should we be crowdsourcing plot ideas, or is this more of a ‘write once, read never’ situation for future generations? Just curious.
That’s a great way to put it! The ‘write once, read never’ aspect is something we definitely need to consider. The potential impact on future generations is a central point of ethical debate surrounding germline editing. Let’s keep this conversation going!
Editor: MedTechNews.Uk
Thank you to our Sponsor Esdebe
The report highlights the challenge of equitable access to genome editing therapies. How can we ensure these potentially transformative treatments don’t exacerbate existing health disparities, especially given the likelihood of high initial costs?
That’s a crucial point! Equitable access is paramount. Exploring tiered pricing models, public funding initiatives, or even open-source research could help bridge the gap and ensure these life-changing therapies reach everyone who needs them, regardless of their background. What other solutions might be viable?
Editor: MedTechNews.Uk
Thank you to our Sponsor Esdebe
Given the potential for misuse outlined in the report, what specific oversight mechanisms beyond current regulatory frameworks could be implemented to prevent malicious applications of genome editing technologies, particularly concerning bioweapons development?