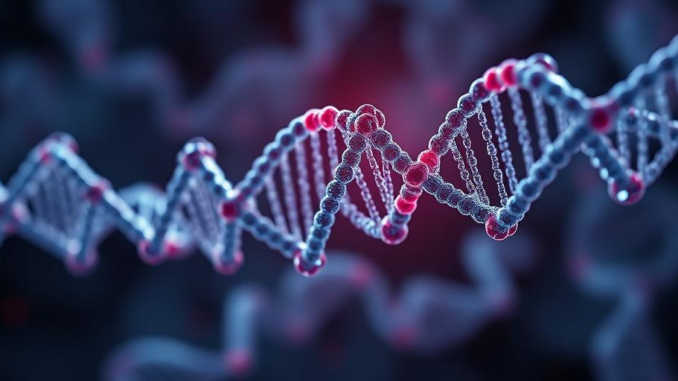
The Evolving Landscape of Mutation: Mechanisms, Consequences, and Therapeutic Interventions
Abstract
Mutations, heritable alterations in the genomic sequence, are fundamental drivers of evolution and disease. This report provides a comprehensive overview of the current understanding of mutation, encompassing the diverse mechanisms by which they arise, the spectrum of their phenotypic consequences, and the strategies being developed to therapeutically address mutation-driven disorders. We delve into the intricacies of spontaneous and induced mutagenesis, highlighting the roles of DNA replication errors, endogenous metabolic processes, and exogenous environmental factors. The report examines various classes of mutations, from single nucleotide variants (SNVs) to large-scale structural rearrangements, and their impact on gene expression, protein function, and cellular processes. A critical analysis of genotype-phenotype correlations is presented, emphasizing the complexities of predicting disease outcomes based solely on mutational profiles. Finally, we explore the evolving landscape of therapeutic interventions targeting mutations, including gene editing technologies, mutation-specific drug development, and personalized medicine approaches. The report concludes by discussing the challenges and future directions in mutation research, emphasizing the need for improved mutation detection technologies, predictive models, and ethical considerations surrounding germline editing.
1. Introduction
Mutation, the alteration of the nucleotide sequence of the genome of an organism, virus, or extrachromosomal DNA, is a ubiquitous phenomenon with profound implications. These changes, arising spontaneously or induced by external factors, are the raw material for evolution, driving adaptation and speciation. However, mutations also underlie a vast array of human diseases, from inherited genetic disorders to acquired cancers. Understanding the mechanisms of mutation, their consequences, and the means to mitigate their effects is therefore a central goal of modern biology and medicine. The concept of a mutation was first introduced by Hugo de Vries in the early 20th century, based on his observations of sudden, heritable variations in the evening primrose (Oenothera lamarckiana). Since then, the field of mutation research has grown exponentially, fueled by advances in molecular biology, genomics, and computational biology. This report aims to provide a comprehensive overview of the current state of mutation research, exploring its multifaceted nature and its relevance to both basic science and clinical practice. It will address the underlying mechanisms of mutagenesis, the diversity of mutational effects, the challenges of genotype-phenotype correlations, and the emerging strategies for therapeutic intervention.
2. Mechanisms of Mutation
Mutations can arise through a variety of mechanisms, broadly categorized as spontaneous or induced. Spontaneous mutations occur naturally during DNA replication, recombination, or repair processes, while induced mutations are caused by exposure to external agents, known as mutagens. Understanding these mechanisms is crucial for developing strategies to prevent or minimize mutation rates.
2.1 Spontaneous Mutagenesis
-
DNA Replication Errors: DNA replication is a remarkably accurate process, but errors can still occur due to the inherent limitations of DNA polymerases. These errors include base misincorporation, where the wrong nucleotide is inserted into the growing DNA strand, and slippage, where DNA polymerase stutters, leading to insertions or deletions (indels) of short sequences. DNA polymerases possess proofreading activity, which helps to correct these errors, but some errors inevitably escape detection and become fixed as mutations. The rate of replication errors varies depending on the DNA polymerase involved and the specific genomic region being replicated. Regions with repetitive sequences are particularly prone to slippage errors.
-
Endogenous Metabolic Processes: Normal metabolic processes can generate reactive oxygen species (ROS) and other reactive metabolites that can damage DNA. For example, oxidative stress can lead to the formation of 8-oxo-guanine (8-oxoG), a modified base that can mispair with adenine during replication, leading to G to T transversions. Hydrolytic reactions, such as deamination of cytosine to uracil, are another source of spontaneous mutations. Uracil in DNA is normally removed by uracil DNA glycosylase (UDG), but if this repair mechanism fails, the uracil can be replicated as thymine, resulting in a C to T transition.
-
Transposable Elements: Transposable elements (TEs), also known as jumping genes, are mobile DNA sequences that can insert themselves into new locations in the genome. TE insertions can disrupt gene function by inserting into coding regions or regulatory elements. They can also cause deletions, inversions, and other chromosomal rearrangements. TEs are a significant source of spontaneous mutations in many organisms, including humans. The activity of TEs is often regulated by epigenetic mechanisms, such as DNA methylation, which can silence TE expression.
2.2 Induced Mutagenesis
-
Chemical Mutagens: A wide range of chemicals can induce mutations by directly damaging DNA or by interfering with DNA replication or repair. Base analogs, such as 5-bromouracil (5-BU), can be incorporated into DNA in place of normal bases, leading to mispairing during replication. Alkylating agents, such as ethyl methanesulfonate (EMS), can add alkyl groups to DNA bases, altering their base-pairing properties. Intercalating agents, such as ethidium bromide, can insert themselves between DNA bases, distorting the DNA structure and interfering with DNA replication and repair. The specific type of mutation induced by a chemical mutagen depends on the chemical’s mechanism of action and the specific DNA sequence being targeted.
-
Radiation: Ionizing radiation, such as X-rays and gamma rays, can directly damage DNA by causing strand breaks and base modifications. Ultraviolet (UV) radiation can cause the formation of pyrimidine dimers, covalent linkages between adjacent pyrimidine bases (thymine or cytosine) on the same DNA strand. These dimers distort the DNA structure and interfere with DNA replication and transcription. The type and extent of DNA damage induced by radiation depend on the dose, the type of radiation, and the presence of protective factors, such as antioxidants.
-
Viral Insertional Mutagenesis: Retroviruses and other viruses can integrate their DNA into the host genome, disrupting gene function or altering gene expression. This process, known as insertional mutagenesis, can lead to cancer if the viral integration site is near an oncogene or tumor suppressor gene. Viral insertional mutagenesis is a significant driver of cancer in some animal models and has also been implicated in human cancers.
3. Types of Mutations and Their Consequences
Mutations can be classified based on their molecular nature and their effects on gene function and phenotype. The consequences of a mutation can range from no detectable effect to complete loss of function or even gain of novel function.
3.1 Single Nucleotide Variants (SNVs)
SNVs are the most common type of genetic variation in the human genome. They involve a change in a single nucleotide base. SNVs can be further classified as transitions (purine to purine or pyrimidine to pyrimidine) or transversions (purine to pyrimidine or vice versa). SNVs in coding regions can be classified as:
-
Missense mutations: Result in a change in the amino acid sequence of the protein. The effect of a missense mutation depends on the nature of the amino acid substitution and its location in the protein. Some missense mutations may have no effect on protein function, while others can significantly alter protein folding, stability, or activity.
-
Nonsense mutations: Introduce a premature stop codon, resulting in a truncated protein. Nonsense mutations typically lead to loss of protein function, although the severity of the effect can depend on the location of the stop codon. Early stop codons are more likely to result in non-functional proteins, while stop codons near the end of the coding sequence may result in proteins with partial function.
-
Silent mutations: Do not change the amino acid sequence of the protein due to the degeneracy of the genetic code. Silent mutations were once considered to be neutral, but it is now recognized that they can affect gene expression and protein folding by influencing mRNA splicing, mRNA stability, or codon usage.
SNVs in non-coding regions can also have significant effects on gene expression by altering regulatory elements, such as promoters, enhancers, and silencers. These mutations can affect the binding of transcription factors, leading to changes in gene transcription. SNVs in splice sites can disrupt mRNA splicing, leading to the production of aberrant mRNA transcripts.
3.2 Insertions and Deletions (Indels)
Indels involve the addition or removal of one or more nucleotides from the DNA sequence. Indels in coding regions can cause frameshift mutations if the number of inserted or deleted nucleotides is not a multiple of three. Frameshift mutations alter the reading frame of the mRNA, leading to a completely different amino acid sequence downstream of the mutation and often resulting in a premature stop codon. Indels in non-coding regions can affect gene expression by disrupting regulatory elements or altering mRNA splicing.
3.3 Structural Variants
Structural variants (SVs) are large-scale alterations in the genome, including deletions, duplications, inversions, and translocations. SVs can encompass entire genes or even multiple genes, leading to dramatic changes in gene dosage or gene structure. Deletions remove a segment of DNA, potentially leading to loss of gene function. Duplications increase the copy number of a DNA segment, which can lead to increased gene expression. Inversions reverse the orientation of a DNA segment, which can disrupt gene function or alter gene expression. Translocations move a DNA segment from one chromosome to another, which can disrupt gene function or create fusion genes.
4. Genotype-Phenotype Correlations
Establishing a clear relationship between genotype (the genetic makeup of an individual) and phenotype (the observable characteristics of an individual) is a major challenge in mutation research. While some mutations have predictable and consistent effects, others exhibit complex and variable phenotypes. Several factors contribute to the complexity of genotype-phenotype correlations, including:
-
Genetic Background: The effects of a mutation can be modified by other genes in the genome. These modifier genes can interact with the mutated gene to enhance or suppress its effects. Genetic background effects can explain why individuals with the same mutation can exhibit different phenotypes.
-
Environmental Factors: Environmental factors, such as diet, lifestyle, and exposure to toxins, can also influence the phenotype. Environmental factors can interact with genetic factors to determine the overall phenotype. For example, individuals with a genetic predisposition to diabetes may only develop the disease if they also have a poor diet and sedentary lifestyle.
-
Epigenetic Modifications: Epigenetic modifications, such as DNA methylation and histone modifications, can alter gene expression without changing the DNA sequence. Epigenetic modifications can be influenced by both genetic and environmental factors and can contribute to phenotypic variation. In some cases, epigenetic modifications can be inherited across generations, leading to transgenerational epigenetic inheritance.
-
Allelic Heterogeneity: Different mutations in the same gene can have different effects on protein function and phenotype. This phenomenon, known as allelic heterogeneity, can make it difficult to predict the phenotype based solely on the gene that is mutated. For example, in cystic fibrosis, different mutations in the CFTR gene can lead to varying degrees of lung disease, pancreatic insufficiency, and other symptoms.
-
Locus Heterogeneity: Mutations in different genes can cause the same or similar phenotypes. This phenomenon, known as locus heterogeneity, can complicate genetic diagnosis and counseling. For example, hearing loss can be caused by mutations in many different genes, each affecting a different aspect of auditory function.
5. Therapeutic Interventions Targeting Mutations
The growing understanding of mutation mechanisms and their consequences has led to the development of a variety of therapeutic interventions aimed at correcting or mitigating the effects of mutations. These interventions can be broadly classified as gene editing, mutation-specific drug development, and personalized medicine approaches.
5.1 Gene Editing Technologies
Gene editing technologies, such as CRISPR-Cas9, offer the potential to directly correct mutations in the genome. CRISPR-Cas9 uses a guide RNA to target a specific DNA sequence, where the Cas9 enzyme introduces a double-strand break. The cell’s own DNA repair mechanisms can then be harnessed to either disrupt the mutated gene or to insert a corrected copy of the gene. Gene editing has shown promising results in preclinical studies for a variety of genetic disorders, including cystic fibrosis, Duchenne muscular dystrophy, and sickle cell anemia. However, gene editing also raises ethical concerns about off-target effects, germline editing, and the potential for unintended consequences.
5.2 Mutation-Specific Drug Development
Mutation-specific drug development focuses on developing drugs that specifically target the protein product of a mutated gene. For example, in cystic fibrosis, modulator drugs, such as ivacaftor and lumacaftor, can improve the function of the mutant CFTR protein. Ivacaftor potentiates the activity of the CFTR protein, while lumacaftor helps to fold the protein correctly and transport it to the cell surface. Mutation-specific drugs offer the advantage of being highly targeted and potentially less toxic than traditional drugs. However, they are only effective for specific mutations and may not be applicable to all patients with the disease.
5.3 Personalized Medicine Approaches
Personalized medicine approaches use an individual’s genetic information to tailor their treatment to their specific needs. This can involve using genetic testing to identify individuals who are at risk for developing a particular disease or to predict their response to a particular drug. Personalized medicine can also involve using genetic information to guide treatment decisions, such as selecting the most appropriate chemotherapy regimen for a patient with cancer. Personalized medicine has the potential to improve the effectiveness and safety of medical treatments. However, it also raises ethical concerns about privacy, discrimination, and the cost of genetic testing.
6. Challenges and Future Directions
Despite the significant progress in mutation research, several challenges remain. These include:
-
Improving Mutation Detection Technologies: Developing more sensitive and accurate methods for detecting mutations is essential for both research and clinical applications. Current sequencing technologies have limitations in detecting certain types of mutations, such as structural variants and mutations in repetitive regions. New technologies, such as long-read sequencing and single-cell sequencing, are being developed to address these limitations.
-
Developing Predictive Models: Developing accurate models for predicting the phenotypic consequences of mutations is a major challenge. These models need to take into account the genetic background, environmental factors, and epigenetic modifications that can influence the phenotype. Machine learning and other computational approaches are being used to develop these predictive models. But I contend that the ability to predict the result of a mutation is less dependent on AI systems and more dependent on complete biological understanding of the processes involved, even at a theoretical level.
-
Addressing Ethical Considerations: The increasing ability to manipulate the genome raises ethical concerns about off-target effects, germline editing, and the potential for unintended consequences. These ethical issues need to be carefully considered and addressed through open and transparent public dialogue and the establishment of appropriate regulatory frameworks.
Future directions in mutation research include:
-
Exploring the Role of Mutations in Aging and Disease: Mutations accumulate throughout life and contribute to the aging process and the development of age-related diseases. Understanding the role of mutations in these processes could lead to new strategies for preventing or treating age-related diseases.
-
Developing New Therapeutic Interventions: New therapeutic interventions are being developed to correct or mitigate the effects of mutations. These include gene editing technologies, mutation-specific drugs, and personalized medicine approaches. These interventions offer the potential to revolutionize the treatment of genetic disorders and other diseases.
-
Promoting Diversity and Inclusion in Mutation Research: It is important to ensure that mutation research is inclusive and representative of all populations. This includes studying mutations in diverse ethnic groups and addressing health disparities that are related to genetic variation.
7. Conclusion
Mutation is a fundamental process that shapes the evolution of life and underlies many human diseases. Understanding the mechanisms of mutation, their consequences, and the means to mitigate their effects is therefore a central goal of modern biology and medicine. The field of mutation research has made significant progress in recent years, leading to the development of new technologies and therapeutic interventions. However, several challenges remain, including improving mutation detection technologies, developing predictive models, and addressing ethical considerations. By continuing to invest in mutation research, we can improve our understanding of the fundamental processes of life and develop new strategies for preventing and treating disease.
References
- Alberts, B., Johnson, A., Lewis, J., Raff, M., Roberts, K., & Walter, P. (2002). Molecular Biology of the Cell (4th ed.). Garland Science.
- Cooper, D. N. (2003). Nature Encyclopedia of the Human Genome. Nature Publishing Group.
- Drake, J. W. (1991). Spontaneous mutation. Annual Review of Genetics, 25(1), 125-146.
- Eyre-Walker, A., & Keightley, P. D. (2007). The rate of spontaneous mutation in animals. Nature, 449(7165), 823-827.
- Griffiths, A. J. F., Miller, J. H., Suzuki, D. T., Lewontin, R. C., & Gelbart, W. M. (2000). An Introduction to Genetic Analysis (7th ed.). W. H. Freeman.
- Lodish, H., Berk, A., Zipursky, S. L., Matsudaira, P., Baltimore, D., & Darnell, J. (2000). Molecular Cell Biology (4th ed.). W. H. Freeman.
- Stenson, P. D., Ball, E. V., Mort, M., Phillips, A. D., Shiel, J. A., Thomas, N. S., … & Cooper, D. N. (2003). Human Gene Mutation Database (HGMD): 2003 update. Human Mutation, 21(6), 577-581.
- Voet, D., Voet, J. G., & Pratt, C. W. (2016). Fundamentals of Biochemistry: Life at the Molecular Level (5th ed.). John Wiley & Sons.
- National Human Genome Research Institute. (n.d.). Retrieved from https://www.genome.gov/
- Strachan T, Read AP. Human Molecular Genetics. 2nd edition. New York: Wiley-Liss; 1999.
- Hwang, T. J., Canver, M. C., Anderson, D. G., Black, J. B., & Doudna, J. A. (2019). Development and use of CRISPR-Cas9 for gene editing. Nature Biotechnology, 37(11), 1233–1242.
- Cutting, G. R. (2015). Cystic fibrosis genetics: from mutation to medicine. Nature Reviews Genetics, 16(1), 45–56.
Be the first to comment