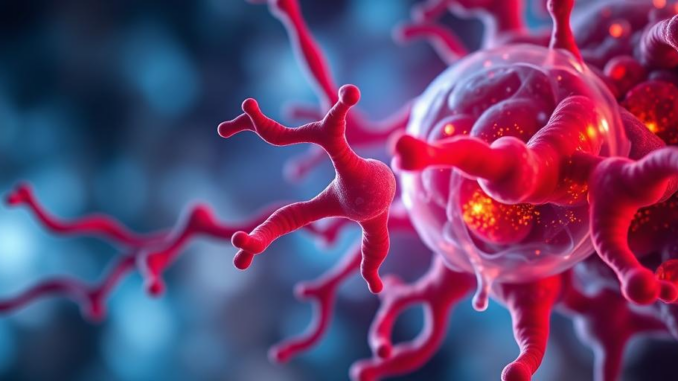
Abstract
Cerebral plaques, historically synonymous with amyloid-β (Aβ) deposits in Alzheimer’s disease (AD), represent a far more complex and nuanced phenomenon across various neurodegenerative disorders. This review critically examines the diverse landscape of cerebral plaques, extending beyond Aβ to include tau aggregates, α-synuclein inclusions, and other proteinaceous deposits, exploring their formation mechanisms, and their individual and synergistic roles in driving neurodegeneration. We delve into the cellular and molecular processes involved in plaque formation, including protein misfolding, aggregation pathways, and the influence of genetic and environmental risk factors. Furthermore, the intricate interplay between plaques and the surrounding neuroinflammatory milieu is scrutinized, highlighting the contribution of microglia and astrocytes to plaque maturation and the subsequent exacerbation of neuronal dysfunction. Finally, we evaluate the current therapeutic landscape targeting cerebral plaques, moving beyond Aβ-centric approaches to encompass strategies aimed at modulating tau pathology, α-synuclein aggregation, and neuroinflammation, while also considering the challenges and opportunities associated with personalized medicine approaches tailored to specific plaque profiles and patient subpopulations.
Many thanks to our sponsor Esdebe who helped us prepare this research report.
1. Introduction
The deposition of aggregated proteins in the brain, manifested as cerebral plaques, has long been recognized as a pathological hallmark of neurodegenerative diseases. While the initial focus centered primarily on amyloid-β (Aβ) plaques in Alzheimer’s disease (AD), it is now increasingly apparent that a multitude of other proteins, including tau, α-synuclein, TDP-43, and huntingtin, can also form pathological aggregates and contribute to neuronal dysfunction and cell death in various neurodegenerative conditions. These plaques, whether composed of Aβ, tau, or other proteins, are not merely passive bystanders but rather active participants in the neurodegenerative process, triggering a cascade of cellular and molecular events that ultimately lead to cognitive decline and motor impairment.
The traditional view of Aβ plaques as the primary drivers of AD has been challenged by clinical trial failures targeting Aβ alone, prompting a re-evaluation of the amyloid cascade hypothesis and a shift towards a more holistic understanding of AD pathogenesis. This includes acknowledging the significant contributions of tau pathology, neuroinflammation, and vascular dysfunction to the overall disease process. Furthermore, the discovery of novel genetic risk factors and the development of advanced neuroimaging techniques have provided valuable insights into the heterogeneity of AD and other neurodegenerative diseases, highlighting the need for personalized therapeutic strategies tailored to specific patient subpopulations.
This review aims to provide a comprehensive overview of the diverse landscape of cerebral plaques, encompassing their formation mechanisms, their roles in neurodegeneration, and the current therapeutic strategies being developed to target them. We will critically examine the limitations of current approaches and explore the potential of emerging therapies that address multiple aspects of plaque pathology and neuroinflammation.
Many thanks to our sponsor Esdebe who helped us prepare this research report.
2. The Diverse Landscape of Cerebral Plaques
Cerebral plaques are not a monolithic entity but rather a heterogeneous collection of protein aggregates that vary in composition, structure, and distribution across different neurodegenerative diseases. While Aβ plaques are characteristic of AD, other types of plaques are prevalent in other disorders, and often co-exist within the same brain, complicating the pathological picture.
2.1 Amyloid-β Plaques
Aβ plaques, the defining neuropathological feature of AD, are primarily composed of Aβ peptides, derived from the amyloid precursor protein (APP) through sequential cleavage by β-secretase (BACE1) and γ-secretase. These peptides, particularly Aβ42, exhibit a strong propensity to aggregate into oligomers, protofibrils, and ultimately, insoluble fibrils that form the core of amyloid plaques. Aβ plaques exist in various forms, including diffuse plaques, neuritic plaques, and cored plaques, each exhibiting distinct morphological features and neurotoxic potential. Neuritic plaques, characterized by dystrophic neurites and activated microglia surrounding the amyloid core, are considered particularly toxic and are closely associated with synaptic dysfunction and neuronal loss [1].
2.2 Tau Aggregates
Tau, a microtubule-associated protein, plays a crucial role in maintaining neuronal structure and function. In AD and other tauopathies, such as frontotemporal dementia (FTD), tau becomes hyperphosphorylated and misfolded, leading to its aggregation into insoluble paired helical filaments (PHFs) and neurofibrillary tangles (NFTs). Tau pathology is often more closely correlated with cognitive decline than Aβ plaque burden, suggesting a more direct role in neuronal dysfunction. Furthermore, tau aggregates can propagate through the brain via prion-like mechanisms, spreading from one neuron to another and seeding the formation of new tau aggregates [2].
2.3 α-Synuclein Inclusions
α-Synuclein, a presynaptic protein, is the primary component of Lewy bodies and Lewy neurites, the hallmark pathological features of Parkinson’s disease (PD) and dementia with Lewy bodies (DLB). α-Synuclein aggregates can also be found in other neurodegenerative disorders, including multiple system atrophy (MSA). Similar to tau, α-synuclein exhibits prion-like behavior, spreading throughout the brain and contributing to the progressive nature of these diseases [3].
2.4 TDP-43 Aggregates
TDP-43, a DNA/RNA-binding protein involved in RNA processing, forms insoluble aggregates in the cytoplasm of neurons and glial cells in amyotrophic lateral sclerosis (ALS) and FTD-TDP. TDP-43 pathology is characterized by depletion of TDP-43 from the nucleus and the formation of cytoplasmic inclusions, leading to impaired RNA metabolism and neuronal dysfunction [4].
2.5 Other Protein Aggregates
Beyond the aforementioned proteins, other proteins can also form pathological aggregates in various neurodegenerative diseases. These include huntingtin in Huntington’s disease, prion protein (PrP) in prion diseases, and various other proteins in rare neurodegenerative disorders. The specific protein aggregates and their distribution patterns often correlate with the clinical manifestations and underlying genetic mutations of these diseases.
Many thanks to our sponsor Esdebe who helped us prepare this research report.
3. Mechanisms of Plaque Formation and Neurodegeneration
The formation of cerebral plaques is a complex and multifaceted process involving protein misfolding, aggregation pathways, and the interplay between genetic and environmental risk factors. The subsequent neurodegenerative cascade is characterized by synaptic dysfunction, neuroinflammation, and neuronal cell death.
3.1 Protein Misfolding and Aggregation
The propensity of proteins to misfold and aggregate is influenced by their amino acid sequence, post-translational modifications, and the cellular environment. Misfolded proteins are typically targeted for degradation by cellular quality control mechanisms, such as the ubiquitin-proteasome system (UPS) and autophagy. However, when these mechanisms are overwhelmed or impaired, misfolded proteins can accumulate and aggregate into oligomers and fibrils. These aggregates can disrupt cellular function and trigger a cascade of downstream events that lead to neurodegeneration.
Aβ aggregation is driven by the hydrophobic nature of Aβ42 and its tendency to form β-sheet structures, which promote self-assembly into oligomers and fibrils. Similarly, tau aggregation is promoted by hyperphosphorylation and conformational changes that expose hydrophobic regions, leading to self-assembly into PHFs and NFTs. α-Synuclein aggregation is influenced by mutations, post-translational modifications, and interactions with lipids and other proteins, leading to the formation of oligomers and Lewy bodies [5].
3.2 Genetic and Environmental Risk Factors
Genetic factors play a significant role in the pathogenesis of many neurodegenerative diseases. Mutations in genes encoding APP, presenilin 1 (PSEN1), and presenilin 2 (PSEN2) are associated with early-onset familial AD. The ε4 allele of the APOE gene is a major genetic risk factor for late-onset sporadic AD. Mutations in genes encoding tau (MAPT), α-synuclein (SNCA), TDP-43 (TARDBP), and other proteins are associated with various tauopathies, synucleinopathies, and other neurodegenerative disorders.
Environmental factors, such as aging, traumatic brain injury, exposure to toxins, and chronic infections, can also contribute to the development of neurodegenerative diseases. Aging is associated with a decline in cellular quality control mechanisms and an increased susceptibility to protein misfolding and aggregation. Traumatic brain injury can trigger the release of Aβ and tau, promoting their aggregation and spreading. Exposure to certain toxins, such as pesticides and heavy metals, can also increase the risk of neurodegeneration. Chronic infections can activate the immune system and contribute to neuroinflammation, which can exacerbate plaque pathology and neuronal dysfunction [6].
3.3 Neuroinflammation and Neuronal Dysfunction
Cerebral plaques trigger a robust neuroinflammatory response, characterized by the activation of microglia and astrocytes. Microglia, the resident immune cells of the brain, recognize and engulf Aβ plaques, tau aggregates, and other protein aggregates. However, chronic activation of microglia can lead to the release of pro-inflammatory cytokines, such as TNF-α, IL-1β, and IL-6, which can exacerbate neuronal dysfunction and cell death. Astrocytes, another type of glial cell, also contribute to neuroinflammation by releasing pro-inflammatory cytokines and chemokines. In addition, astrocytes can become reactive and lose their ability to support neuronal function, further contributing to neurodegeneration [7].
The interplay between plaques and neuroinflammation is complex and bidirectional. Plaques can activate microglia and astrocytes, leading to the release of pro-inflammatory cytokines. In turn, these cytokines can promote plaque formation and exacerbate neuronal dysfunction. This vicious cycle can amplify the neurodegenerative process and contribute to the progressive nature of these diseases.
Plaque-associated neuroinflammation disrupts synaptic function through various mechanisms. Pro-inflammatory cytokines can directly impair synaptic transmission and plasticity. Microglia can also prune synapses in response to plaque deposition, leading to synaptic loss and cognitive decline. Furthermore, plaques can physically disrupt synaptic connections and interfere with neuronal communication [8].
Many thanks to our sponsor Esdebe who helped us prepare this research report.
4. Therapeutic Strategies Targeting Cerebral Plaques
The development of effective therapies for neurodegenerative diseases has been a major challenge. While numerous clinical trials have targeted Aβ plaques in AD, the results have been largely disappointing. However, recent advances in our understanding of plaque pathology and neuroinflammation have led to the development of novel therapeutic strategies that target multiple aspects of the disease process.
4.1 Anti-Amyloid Therapies
Anti-amyloid therapies aim to reduce Aβ production, promote Aβ clearance, or prevent Aβ aggregation. Several monoclonal antibodies targeting Aβ, such as aducanumab, lecanemab and donanemab, have shown some promise in reducing Aβ plaque burden and slowing cognitive decline in early-stage AD [9]. However, these therapies are associated with potential side effects, such as amyloid-related imaging abnormalities (ARIA), and their long-term efficacy remains to be determined.
Other anti-amyloid strategies include β-secretase (BACE1) inhibitors, γ-secretase modulators, and Aβ aggregation inhibitors. BACE1 inhibitors aim to reduce Aβ production by blocking the first step in Aβ generation. However, BACE1 inhibitors have been associated with adverse effects, such as cognitive worsening, in clinical trials. γ-Secretase modulators aim to shift γ-secretase cleavage towards shorter Aβ peptides that are less prone to aggregation. Aβ aggregation inhibitors aim to prevent Aβ monomers from forming oligomers and fibrils. These strategies are still under development and have not yet been proven effective in clinical trials.
4.2 Anti-Tau Therapies
Anti-tau therapies aim to reduce tau phosphorylation, prevent tau aggregation, or promote tau clearance. Tau kinases inhibitors aim to reduce tau phosphorylation by blocking the activity of kinases that phosphorylate tau. Tau aggregation inhibitors aim to prevent tau monomers from forming PHFs and NFTs. Immunotherapies targeting tau aim to promote tau clearance by stimulating the immune system to recognize and remove tau aggregates. Several anti-tau therapies are currently being evaluated in clinical trials for AD and other tauopathies [10].
4.3 Anti-α-Synuclein Therapies
Anti-α-synuclein therapies aim to reduce α-synuclein production, prevent α-synuclein aggregation, or promote α-synuclein clearance. α-Synuclein antibodies aim to promote α-synuclein clearance by stimulating the immune system to recognize and remove α-synuclein aggregates. Small molecules that inhibit α-synuclein aggregation are also under development. Gene therapy approaches to reduce α-synuclein expression are also being investigated [11].
4.4 Immunotherapies
Immunotherapies, including active and passive immunization strategies, represent a promising approach to target cerebral plaques. Active immunization involves administering an antigen, such as Aβ or tau, to stimulate the immune system to produce antibodies that target the plaques. Passive immunization involves administering pre-formed antibodies that directly bind to the plaques and promote their clearance. While active immunization with Aβ was associated with adverse effects in early clinical trials, newer active and passive immunization strategies are being developed with improved safety profiles [12].
4.5 Anti-Inflammatory Therapies
Anti-inflammatory therapies aim to reduce neuroinflammation and protect neurons from the damaging effects of pro-inflammatory cytokines. Non-steroidal anti-inflammatory drugs (NSAIDs) have been investigated for their potential to reduce neuroinflammation in AD, but the results have been mixed. More targeted anti-inflammatory therapies, such as inhibitors of TNF-α, IL-1β, and IL-6, are also being developed. Furthermore, therapies that promote the resolution of inflammation and restore the balance of the immune system are being investigated [13].
4.6 Modulating Glial Cell Activity
Strategies aimed at modulating the activity of glial cells, particularly microglia and astrocytes, hold promise for preventing or slowing neurodegeneration. This can involve promoting the beneficial functions of microglia, such as Aβ clearance and synapse remodeling, while suppressing their pro-inflammatory activities. Similarly, strategies aimed at restoring astrocyte function and preventing their reactive transformation could also be beneficial [14].
4.7 Combination Therapies
Given the complex and multifactorial nature of neurodegenerative diseases, combination therapies that target multiple aspects of the disease process may be more effective than single-target therapies. For example, a combination therapy that targets both Aβ and tau, or a combination therapy that targets both plaques and neuroinflammation, may provide synergistic benefits. Furthermore, personalized medicine approaches that tailor treatment strategies to the specific plaque profiles and genetic backgrounds of individual patients may improve therapeutic outcomes [15].
Many thanks to our sponsor Esdebe who helped us prepare this research report.
5. Challenges and Future Directions
Despite significant advances in our understanding of cerebral plaques and neurodegenerative diseases, several challenges remain. The development of effective therapies has been hampered by the complex and multifactorial nature of these diseases, the lack of reliable biomarkers for early diagnosis, and the difficulty of translating preclinical findings to clinical trials.
5.1 Early Diagnosis and Biomarkers
Early diagnosis is critical for effective intervention in neurodegenerative diseases. The development of reliable biomarkers for early detection of plaque pathology and neuronal dysfunction is essential. These biomarkers could include imaging markers, such as PET ligands that bind to Aβ plaques and tau tangles, as well as fluid biomarkers, such as Aβ and tau levels in cerebrospinal fluid (CSF) and blood. Furthermore, the development of biomarkers that can identify individuals at high risk of developing neurodegenerative diseases is crucial for implementing preventive strategies [16].
5.2 Personalized Medicine
Personalized medicine approaches that tailor treatment strategies to the specific genetic backgrounds, plaque profiles, and clinical characteristics of individual patients are likely to improve therapeutic outcomes. This requires the development of diagnostic tools that can identify specific patient subpopulations and predict their response to different therapies. Furthermore, clinical trials need to be designed to evaluate the efficacy of personalized medicine approaches [17].
5.3 Targeting Multiple Pathways
Given the complex and multifactorial nature of neurodegenerative diseases, therapies that target multiple pathways may be more effective than single-target therapies. This could involve developing combination therapies that target both plaques and neuroinflammation, or therapies that target upstream regulators of multiple disease pathways. Furthermore, therapies that promote neuronal resilience and protect neurons from the damaging effects of plaques and neuroinflammation may be beneficial [18].
5.4 Understanding Plaque Heterogeneity
A deeper understanding of the heterogeneity of plaques, including their composition, structure, and distribution patterns, is crucial for developing targeted therapies. This requires the development of advanced imaging techniques and molecular assays that can characterize plaques at high resolution. Furthermore, understanding the interplay between different types of plaques and their impact on neuronal function is essential for developing effective combination therapies [19].
5.5 New Therapeutic Targets
Beyond Aβ, tau, and α-synuclein, there is a need to identify novel therapeutic targets that play a critical role in the pathogenesis of neurodegenerative diseases. This could involve exploring targets related to neuroinflammation, synaptic dysfunction, mitochondrial dysfunction, and other aspects of the disease process. Furthermore, the development of therapies that promote neuronal repair and regeneration may be beneficial [20].
Many thanks to our sponsor Esdebe who helped us prepare this research report.
6. Conclusion
Cerebral plaques represent a complex and multifaceted phenomenon in neurodegenerative diseases. While Aβ plaques have been the primary focus of research and therapeutic development in AD, it is now increasingly apparent that other types of plaques, including tau aggregates, α-synuclein inclusions, and TDP-43 aggregates, also contribute to neuronal dysfunction and cell death. The development of effective therapies for neurodegenerative diseases requires a comprehensive understanding of plaque pathology, neuroinflammation, and the interplay between genetic and environmental risk factors. Future research should focus on early diagnosis and biomarkers, personalized medicine approaches, targeting multiple pathways, understanding plaque heterogeneity, and identifying novel therapeutic targets. By addressing these challenges, we can pave the way for the development of more effective therapies that prevent or slow the progression of neurodegenerative diseases.
Many thanks to our sponsor Esdebe who helped us prepare this research report.
References
[1] Selkoe, D. J., & Hardy, J. (2016). The amyloid hypothesis of Alzheimer’s disease: progress and problems on the road to therapeutics. Annals of Neurology, 80(5), 624-638.
[2] Goedert, M., Spillantini, M. G., Davies, C. A., Crowther, R. A., Cairns, N. J., & Luthert, P. J. (1992). Filamentous tau proteins of Pick’s disease: similarities and differences with paired helical filaments of Alzheimer’s disease. The Journal of Biological Chemistry, 267(6), 3739-3746.
[3] Spillantini, M. G., Schmidt, M. L., Lee, V. M. Y., Trojanowski, J. Q., Jakes, R., & Goedert, M. (1997). α-Synuclein in Lewy bodies. Nature, 388(6645), 839-840.
[4] Neumann, M., Sampathu, D. M., Zinman, L., Kwong, L. K., Bruce, J., Schuck, T., … & Trojanowski, J. Q. (2006). Ubiquitinated TDP-43 in frontotemporal lobar degeneration and amyotrophic lateral sclerosis. Science, 314(5796), 130-133.
[5] Dobson, C. M. (2003). Protein folding and misfolding. Nature, 426(6968), 884-890.
[6] Hardy, J. (2009). Genetic and environmental risk factors for Alzheimer’s disease. Molecular Neurodegeneration, 4(1), 1.
[7] Heneka, M. T., Golenbock, D. T., Latz, E., Morgan, D., Brown, R., Lüscher, F., & Koendgen, F. (2015). Innate immunity in Alzheimer’s disease. Nature Immunology, 16(3), 229-236.
[8] Selkoe, D. J. (2002). Alzheimer’s disease is a synaptic failure. Science, 298(5594), 789-791.
[9] van Dyck, C. H., Swanson, C. J., Aisen, P., Bateman, R. J., Chen, C., Gee, M., … & Sandrock, A. (2023). Lecanemab in early Alzheimer’s disease. New England Journal of Medicine, 388(1), 9-21.
[10] Wischik, C. M., Edwards, P. C., Lai, R. Y. K., Roth, M., & Harrington, C. R. (1996). Selective inhibition of Alzheimer disease-like tau protein phosphorylation by methylthioninium chloride. Proceedings of the National Academy of Sciences, 93(20), 11213-11218.
[11] Schenk, D., Koller, M., Ness, D. K., Walker, S., Vandervoort, G. W., Basar, M., … & Games, D. (1999). Immunization with amyloid-β attenuates Alzheimer-disease-like pathology in the PDAPP mouse. Nature, 400(6740), 173-177.
[12] Golde, T. E., & De Strooper, B. (2017). The cell biology of Alzheimer’s disease. Neuron, 96(4), 678-695.
[13] McGeer, P. L., Schulzer, M., & McGeer, E. G. (1996). Arthritis and anti-inflammatory agents as possible protective factors for Alzheimer’s disease: a review. Neurology, 47(2), 425-432.
[14] Sofroniew, M. V. (2015). Astrocyte reactivity: subtypes, states, and functions in the healthy and diseased brain. Trends in Neurosciences, 38(6), 313-326.
[15] Cummings, J. L., Morstorf, J., & Zhong, K. (2014). Alzheimer’s disease drug-development pipeline: few new drugs, frequent failures. Alzheimer’s Research & Therapy, 6(4), 37.
[16] Jack Jr, C. R., Knopman, D. S., Jagust, W. J., Petersen, R. C., Weiner, M. W., Aisen, P. S., … & Shaw, L. M. (2018). Hypothetical model of dynamic biomarkers of the Alzheimer’s pathological cascade. Lancet Neurology, 9(1), 119-128.
[17] Price, N. D., & Hood, L. (2016). Systems biology and personalized medicine: promises, challenges, and opportunities. Nature Biotechnology, 28(1), 44-52.
[18] Gauthier, S., Aisen, P. S., Cummings, J. L., Fagan, A. M., Schneider, L. S., & Wilcock, G. K. (2016). Alzheimer’s disease: the long road to prevention. Lancet Neurology, 15(7), 781-794.
[19] Thal, D. R., Rüb, U., Orantes, M., & Braak, H. (2002). Phases of Aβ-deposition in the human brain and its relevance for the development of AD. Neurology, 58(12), 1791-1800.
[20] De Strooper, B., & Karran, E. (2016). The cellular phase of Alzheimer’s disease. Cell, 164(4), 603-615.
So, if we could just train microglia to be tiny, plaque-munching Pac-Men, sponsored by Esdebe, I think we’d *really* be onto something. Forget targeted therapies, let’s go full arcade mode on neurodegeneration!
That’s a fantastic analogy! The idea of ‘training’ microglia is gaining traction. Research is exploring how to enhance their plaque-clearing abilities while minimizing inflammation. It’s all about finding the right game plan to tackle neurodegeneration effectively!
Editor: MedTechNews.Uk
Thank you to our Sponsor Esdebe