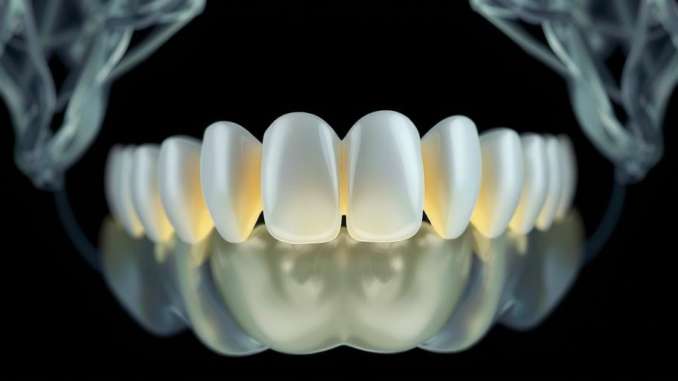
Abstract
Implantology, encompassing orthopedic, dental, and other biomedical applications, is rapidly evolving, driven by advancements in materials science, manufacturing technologies, and computational modeling. This review provides a comprehensive overview of the current state-of-the-art in implant materials, focusing on metals, ceramics, polymers, and composites, with a particular emphasis on surface modifications and coatings designed to enhance biocompatibility and osseointegration. Furthermore, we delve into advanced manufacturing techniques, including additive manufacturing (3D printing), precision machining, and biofabrication, exploring their potential to create customized implants with tailored mechanical properties and biological functionalities. Finally, we address the long-term performance and potential complications associated with various implant designs and materials, highlighting emerging strategies for monitoring implant health and predicting failure. This review aims to provide experts in the field with a critical assessment of current trends and a roadmap for future research directions in implantology.
Many thanks to our sponsor Esdebe who helped us prepare this research report.
1. Introduction
Implantology represents a cornerstone of modern medicine, offering solutions for restoring function and improving the quality of life for individuals suffering from a wide range of conditions, from orthopedic injuries to dental deficiencies. The success of an implant depends on a complex interplay of factors, including the material properties, implant design, surgical technique, and the patient’s physiological response. While traditional implant designs have proven effective in many cases, the pursuit of personalized medicine has spurred the development of customized implants tailored to individual patient needs. This customization is facilitated by advancements in imaging technologies, computational modeling, and advanced manufacturing techniques. A key consideration is the biomaterial selection. While well-established materials like titanium alloys and stainless steels continue to dominate the market, research is increasingly focused on novel materials with enhanced biocompatibility, improved mechanical properties, and inherent antimicrobial characteristics. Furthermore, surface modifications and coatings play a crucial role in modulating the host-implant interaction, promoting osseointegration, and minimizing the risk of infection.
This review aims to provide an in-depth analysis of the latest advancements in implant materials and manufacturing technologies, offering a critical perspective on the challenges and opportunities that lie ahead. We will examine the key material classes used in implantology, explore the various manufacturing processes employed to create both standard and customized implants, and discuss the long-term performance and potential complications associated with these devices. Furthermore, we will explore the emerging field of biofabrication and its potential to revolutionize implant design and function.
Many thanks to our sponsor Esdebe who helped us prepare this research report.
2. Implant Materials: From Metals to Bioactive Composites
2.1 Metallic Implants
Metals have long been the workhorse of implantology, owing to their high strength, ductility, and fatigue resistance. Titanium alloys, particularly Ti-6Al-4V, remain the most widely used metallic implant materials due to their excellent biocompatibility and corrosion resistance [1]. However, concerns regarding the release of vanadium and aluminum ions, which can potentially lead to adverse health effects, have driven research towards alternative titanium alloys, such as Ti-6Al-7Nb and Ti-13Nb-13Zr [2]. These alloys offer comparable mechanical properties to Ti-6Al-4V but with improved biocompatibility.
Stainless steels, specifically 316L stainless steel, are another class of metallic implant materials, particularly for temporary implants and applications where high strength is required at a lower cost. However, their lower corrosion resistance compared to titanium alloys makes them less suitable for long-term implantation [3].
Cobalt-chromium alloys, such as CoCrMo, are primarily used in orthopedic implants, particularly in hip and knee replacements, due to their high wear resistance and fatigue strength [4]. However, concerns about metal ion release and potential allergic reactions have led to the development of alternative bearing surfaces, such as ceramics and highly cross-linked polyethylene. Furthermore, research has focused on developing porous metallic implants to enhance osseointegration. Techniques such as powder metallurgy and additive manufacturing are used to create porous structures with interconnected pores that allow for bone ingrowth, leading to improved implant fixation [5].
2.2 Ceramic Implants
Ceramics offer excellent biocompatibility and wear resistance, making them attractive for implant applications. Alumina (Al2O3) and zirconia (ZrO2) are the most commonly used ceramic materials in implantology. Alumina is known for its high wear resistance and is often used as a bearing surface in hip replacements [6]. Zirconia, particularly yttria-stabilized zirconia (Y-TZP), exhibits higher fracture toughness compared to alumina and is gaining popularity as a dental implant material [7].
Bioactive ceramics, such as hydroxyapatite (HA) and tricalcium phosphate (TCP), are capable of forming a direct chemical bond with bone, promoting rapid osseointegration [8]. HA coatings are commonly applied to metallic implants to enhance their biocompatibility and accelerate bone ingrowth. However, the long-term stability of HA coatings remains a concern, as they can degrade over time. The degradation products can sometimes cause inflammation.
2.3 Polymeric Implants
Polymers offer versatility in terms of their mechanical properties and degradation rates, making them suitable for a wide range of implant applications. Polyetheretherketone (PEEK) is a high-performance polymer that is increasingly used in orthopedic and spinal implants due to its biocompatibility, radiolucency, and mechanical properties that are similar to bone [9]. Polymethylmethacrylate (PMMA) is a widely used bone cement that provides immediate fixation of implants to bone. However, its lack of osseointegration and potential for loosening limit its long-term use [10].
Biodegradable polymers, such as polylactic acid (PLA), polyglycolic acid (PGA), and their copolymers, are used in temporary implants, such as sutures, screws, and drug delivery systems [11]. These polymers degrade over time, eliminating the need for a second surgery to remove the implant. The degradation products are generally non-toxic and are metabolized by the body.
2.4 Composite Implants
Composite materials combine the advantages of different material classes, allowing for the creation of implants with tailored properties. Carbon fiber-reinforced polymers (CFRP) offer high strength-to-weight ratios and are used in orthopedic implants, particularly for load-bearing applications [12]. However, the potential for carbon fiber debris to cause inflammation remains a concern. Polymer-ceramic composites, such as PEEK reinforced with HA, combine the biocompatibility and osseointegrative properties of ceramics with the mechanical properties of polymers [13]. These composites offer a promising alternative to traditional implant materials.
2.5 Surface Modifications and Coatings
Surface modifications and coatings play a critical role in modulating the host-implant interaction and improving implant performance. Techniques such as grit blasting, acid etching, and plasma spraying are used to create rough surfaces that promote cell adhesion and osseointegration [14]. Bioactive coatings, such as HA and titanium dioxide (TiO2), enhance biocompatibility and accelerate bone ingrowth [15]. Antimicrobial coatings, such as silver and antibiotics, reduce the risk of infection [16]. The design of such coatings needs careful consideration to prevent resistance or allergic reaction.
Many thanks to our sponsor Esdebe who helped us prepare this research report.
3. Advanced Manufacturing Technologies for Implant Fabrication
3.1 Additive Manufacturing (3D Printing)
Additive manufacturing (AM), also known as 3D printing, has revolutionized implant fabrication by enabling the creation of complex geometries and customized designs [17]. AM techniques, such as selective laser melting (SLM), electron beam melting (EBM), and fused deposition modeling (FDM), are used to fabricate implants from a variety of materials, including metals, ceramics, and polymers [18]. SLM and EBM are particularly well-suited for manufacturing metallic implants with complex internal structures, such as porous scaffolds for bone ingrowth [19]. FDM is commonly used for printing polymeric implants and customized surgical guides. The resolution and accuracy of AM processes are constantly improving, allowing for the fabrication of implants with increasingly intricate details.
The ability to create patient-specific implants using AM offers significant advantages over traditional manufacturing methods. Customized implants can be designed to perfectly match the patient’s anatomy, improving implant fit, stability, and functional outcome. Furthermore, AM allows for the incorporation of complex features, such as interconnected pores and graded material properties, which can enhance osseointegration and load transfer [20]. AM also enables the rapid prototyping of new implant designs, accelerating the development process and allowing for greater design flexibility. However, the mechanical properties of AM-fabricated implants can be influenced by process parameters, such as laser power, scan speed, and layer thickness, requiring careful optimization and quality control.
3.2 Precision Machining
Precision machining, including milling, turning, and grinding, remains an essential manufacturing technique for implant fabrication [21]. CNC machining offers high precision and dimensional accuracy, making it suitable for producing implants with tight tolerances and smooth surface finishes. Precision machining is often used in conjunction with AM to refine the surface finish and dimensional accuracy of 3D-printed implants. Furthermore, precision machining is used to create complex features, such as threads and grooves, that are difficult to achieve with AM alone.
3.3 Biofabrication
Biofabrication is an emerging field that combines principles of tissue engineering and additive manufacturing to create functional biological constructs, including cell-laden scaffolds and tissue-engineered implants [22]. Biofabrication techniques, such as bioprinting and electrospinning, are used to deposit cells, biomaterials, and growth factors in a spatially controlled manner to create three-dimensional tissue structures [23]. Biofabricated implants hold great promise for regenerative medicine applications, as they can potentially integrate seamlessly with the host tissue and promote tissue regeneration. However, the field of biofabrication is still in its early stages, and significant challenges remain in terms of scaling up the manufacturing process and ensuring the long-term viability and functionality of biofabricated implants.
Many thanks to our sponsor Esdebe who helped us prepare this research report.
4. Long-Term Performance and Potential Complications
4.1 Mechanical Failure
Mechanical failure, including fracture, fatigue, and wear, is a major concern in implantology [24]. Implant fracture can occur due to overloading, material defects, or improper surgical technique. Fatigue failure can result from cyclic loading over time, leading to crack initiation and propagation. Wear can occur at articulating surfaces, such as in hip and knee replacements, generating wear debris that can cause inflammation and osteolysis [25]. The risk of mechanical failure can be minimized by careful material selection, implant design optimization, and adherence to proper surgical techniques.
4.2 Infection
Implant-associated infection is a serious complication that can lead to implant loosening, bone loss, and the need for revision surgery [26]. Bacteria can adhere to the implant surface and form biofilms, which are resistant to antibiotics and host immune defenses. Strategies for preventing implant-associated infection include the use of antimicrobial coatings, the incorporation of antibiotics into the implant material, and the implementation of strict surgical protocols [27].
4.3 Osseointegration Failure
Osseointegration is the direct structural and functional connection between bone and the implant surface. Failure of osseointegration can lead to implant instability and loss of fixation [28]. Factors that can affect osseointegration include the implant material, surface properties, surgical technique, and the patient’s bone quality. Strategies for promoting osseointegration include the use of bioactive coatings, the creation of porous implant surfaces, and the application of growth factors [29].
4.4 Metal Ion Release and Hypersensitivity
Metal ion release from metallic implants can lead to local tissue reactions and systemic hypersensitivity [30]. The release of metal ions can trigger inflammation, osteolysis, and allergic reactions. Strategies for minimizing metal ion release include the use of biocompatible alloys, surface modifications, and coatings. In patients with known metal allergies, non-metallic implants, such as ceramics and polymers, may be considered.
4.5 Implant Loosening
Implant loosening is a common complication, particularly in orthopedic implants, that can lead to pain, instability, and the need for revision surgery [31]. Implant loosening can be caused by mechanical failure, infection, osteolysis, or inadequate osseointegration. Strategies for preventing implant loosening include careful implant design, proper surgical technique, and the optimization of osseointegration.
Many thanks to our sponsor Esdebe who helped us prepare this research report.
5. Future Directions and Concluding Remarks
The field of implantology is rapidly evolving, driven by advancements in materials science, manufacturing technologies, and computational modeling. Future research directions include the development of novel biomaterials with enhanced biocompatibility, improved mechanical properties, and inherent antimicrobial characteristics. The use of additive manufacturing will continue to expand, enabling the creation of customized implants with tailored mechanical properties and biological functionalities. The field of biofabrication holds great promise for creating tissue-engineered implants that can seamlessly integrate with the host tissue and promote tissue regeneration.
Furthermore, the development of advanced monitoring technologies, such as wireless sensors and imaging techniques, will enable the early detection of implant failure and the optimization of treatment strategies. Computational modeling will play an increasingly important role in implant design and performance prediction, allowing for the development of more durable and biocompatible implants. Finally, a greater emphasis on personalized medicine will lead to the development of implants that are tailored to the individual patient’s needs, improving implant outcomes and patient satisfaction.
In conclusion, implantology is a dynamic and rapidly evolving field that offers significant opportunities to improve the quality of life for individuals suffering from a wide range of conditions. By continuing to push the boundaries of materials science, manufacturing technologies, and computational modeling, we can create implants that are more durable, biocompatible, and effective.
Many thanks to our sponsor Esdebe who helped us prepare this research report.
References
[1] Geetha, M., Singh, A. K., Asokamani, R., & Gogia, A. K. (2009). Ti based biomaterials, the ultimate choice for orthopaedic implants – A review. Progress in Materials Science, 54(3), 397-425.
[2] Niinomi, M. (2008). Mechanical biocompatibilities of titanium alloys for biomedical applications. Journal of the Mechanical Behavior of Biomedical Materials, 1(1), 30-42.
[3] Davis, J. R. (2000). Stainless steels. ASM International.
[4] Levine, D. L. (2006). Cobalt-chromium alloys for medical applications. ASM International Handbook, 2, 57-73.
[5] Bobyn, J. D., Pilliar, R. M., Cameron, H. U., & Weatherly, G. C. (1980). The optimum pore size for the fixation of porous-surfaced metal implants by the ingrowth of bone. Clinical Orthopaedics and Related Research, 150, 263-270.
[6] Willmann, G. (1999). Alumina in hip endoprostheses. Clinical Materials, 20(3-4), 149-163.
[7] Piconi, C., & Maccauro, G. (1999). Zirconia as a ceramic for orthopaedic and dental implants: review of the literature. Biomaterials, 20(1), 1-25.
[8] Hench, L. L. (1998). Bioceramics. Journal of the American Ceramic Society, 81(7), 1705-1728.
[9] Kurtz, S. M., Devine, J. N., & Gilbert, J. L. (2007). PEEK biomaterials handbook. William Andrew Publishing.
[10] Lewis, G. (1997). Properties of acrylic bone cement: state of the art review. Journal of Biomedical Materials Research, 38(2), 155-182.
[11] Nair, L. S., & Laurencin, C. T. (2007). Biodegradable polymers as biomaterials. Progress in Polymer Science, 32(8-9), 762-798.
[12] Niinomi, M. (2015). Metallic biomaterials. Springer Science & Business Media.
[13] Khorasani, M., Ghasemi, A. H., Leary, M., Cochrane, R., Dalgarno, P., & Baroutaji, A. (2022). Additive manufacturing of biomedical implants: A review of materials, techniques, and challenges. Journal of Materials Research and Technology, 16, 2068-2095.
[14] Anselme, K. (2000). Osteoblast adhesion on biomaterials. Biomaterials, 21(7), 667-681.
[15] Habibi, S., Yunus, R., Ahmad, Z. A., & Khalil, K. A. (2010). Physical and mechanical properties of plasma sprayed hydroxyapatite coatings on titanium alloy for biomedical applications: a review. Journal of Alloys and Compounds, 494(1-2), 78-91.
[16] Hetrick, E. M., & Schoenfisch, M. H. (2006). Reducing implant-related infections: active release strategies. Chemical Society Reviews, 35(3), 278-289.
[17] Ligon, S. C., Liska, R., Stampfl, J., Gurr, M., & Mülhaupt, R. (2017). Polymers for 3D printing and customized additive manufacturing. Chemical Reviews, 117(15), 10212-10290.
[18] Ngo, T. D., Kashani, A., Imbalzano, G., Nguyen, K. T. Q., & Hui, D. (2018). Additive manufacturing (3D printing): A review of materials, methods, applications and challenges. Composites Part B: Engineering, 143, 172-196.
[19] DebRoy, T., Wei, H. L., Zuback, J. S., Mukherjee, T., Elmer, J. W., Milewski, J. O., … & Zhang, W. (2018). Additive manufacturing of metallic components – Process, structure and properties. Progress in Materials Science, 92, 112-224.
[20] Arabnejad, S., Johnston, R. B., Tanzer, M., & Pasini, D. (2013). Fully porous 3D-printed titanium femoral stems for cementless hip arthroplasty: Mechanical behavior and in vitro osseointegration. Journal of Biomedical Materials Research Part A, 101(12), 3560-3569.
[21] Boothroyd, G., Dewhurst, P., & Knight, W. (2010). Product design for manufacture and assembly. CRC press.
[22] Murphy, S. V., & Atala, A. (2014). 3D bioprinting of tissues and organs. Nature Biotechnology, 32(8), 773-785.
[23] Groll, J., Boland, T., Blunk, T., Burdick, J. A., Cho, D. W., Dalton, P. D., … & Woodfield, T. B. F. (2016). Biofabrication: a manufacturing revolution is approaching. Advanced Materials, 28(13), 2337-2368.
[24] Jacobs, J. J., Gilbert, J. L., & Urban, R. M. (1998). Corrosion of metal orthopaedic implants. Journal of Bone and Joint Surgery-American Volume, 80(2), 268-282.
[25] Ingham, E., & Fisher, J. (2000). The effects of wear debris from total joint replacements. Proceedings of the Institution of Mechanical Engineers, Part H: Journal of Engineering in Medicine, 214(1), 21-37.
[26] Trampuz, A., Widmer, A. F., Zimmerli, W. (2006). Biofilm infections: general and local treatment strategies. Injury, Int. J. Care Injured 37S, S103–S113
[27] Campoccia, D., Montanaro, L., Arciola, C. (2013). A review of the clinical implications of infections caused by biomaterial-associated biofilms. Biomaterials 34, 3315–3330
[28] Albrektsson, T., Branemark, P. I., Hansson, H. A., & Lindstrom, J. (1981). Osseointegrated titanium implants. Requirements for ensuring a long-lasting, direct bone anchorage in man. Acta Orthopaedica Scandinavica, 52(2), 155-170.
[29] Davies, J. E. (2003). Bone bonding at natural and biomaterial surfaces. Biomaterials, 24(13), 2101-2131.
[30] Hallab, N. J., Jacobs, J. J., Skipor, A., Black, J., & Wooley, P. (2001). Systemic metal protein binding associated with total hip arthroplasty. Journal of Biomedical Materials Research, 57(3), 330-339.
[31] Harris, W. H. (2001). Osteolysis and particle disease in total hip arthroplasty: etiology, prevention, and treatment. Journal of Bone and Joint Surgery-American Volume, 83(1), 143-165.
Biofabrication for tissue-engineered implants, huh? Sounds great in theory, but how do you plan to keep those cells alive and kicking *after* implantation, especially when blood supply is questionable? Asking for a friend…who’s a rapidly dying tissue scaffold.
That’s a fantastic point about maintaining cell viability post-implantation! We’re exploring several strategies, including incorporating growth factors directly into the scaffold and designing porous structures to promote rapid vascularization. It’s definitely a key challenge, and innovative solutions are crucial for the success of biofabricated implants.
Editor: MedTechNews.Uk
Thank you to our Sponsor Esdebe
So, we’re 3D printing body parts now? I’m picturing a future where spare organs are like printer cartridges, just pop ’em in! Though, knowing my luck, I’d probably get a “low ink” warning during surgery.
That’s a hilarious analogy! Imagine upgrading your skeletal system like installing new RAM. On a serious note, 3D printing is pushing boundaries in creating customized implants that perfectly fit individual needs and improve overall performance. It is quite an exciting time for this technology!
Editor: MedTechNews.Uk
Thank you to our Sponsor Esdebe