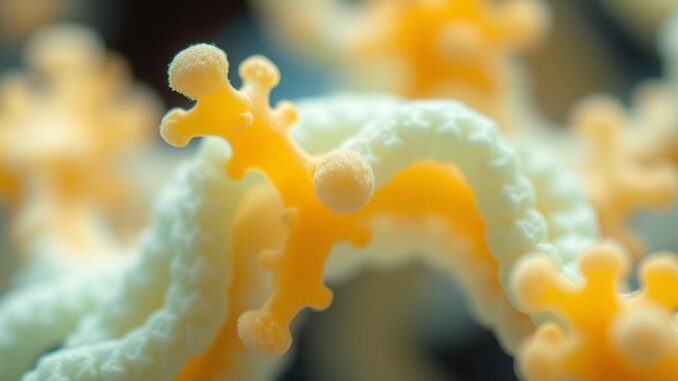
Abstract
Scaffolds play a crucial role in tissue engineering and regenerative medicine, serving as a three-dimensional template for cell attachment, proliferation, differentiation, and ultimately, the formation of functional tissues. While 3D bioprinting has garnered significant attention as a powerful scaffold fabrication technique, it represents only one facet of a complex and rapidly evolving field. This research report provides a comprehensive overview of advanced scaffolding strategies, encompassing a broad range of biomaterials, fabrication techniques, design considerations, and challenges. We delve into the nuances of biomaterial selection, exploring the trade-offs between natural and synthetic polymers, and their impact on biocompatibility, biodegradability, and mechanical properties. We critically assess various fabrication methods, including electrospinning, freeze-drying, and microfluidics, alongside bioprinting, highlighting their respective strengths and limitations. Furthermore, we address the crucial aspects of scaffold design, including porosity, pore size distribution, and surface modification, tailored to specific tissue engineering applications. A significant portion of this report focuses on the persistent challenges in vascularization and strategies for improving scaffold integration with the host tissue. Finally, we discuss emerging trends and future directions in scaffold technology, emphasizing the need for personalized scaffolds, smart biomaterials, and advanced characterization techniques to accelerate the translation of engineered tissues into clinical applications.
Many thanks to our sponsor Esdebe who helped us prepare this research report.
1. Introduction
The promise of tissue engineering and regenerative medicine lies in the ability to repair, replace, or regenerate damaged tissues and organs, offering potential solutions for a wide range of debilitating conditions. Scaffolds, acting as temporary extracellular matrices (ECMs), are fundamental components in this endeavor. They provide structural support, guide cell behavior, and facilitate tissue formation in vitro and in vivo. The ideal scaffold should possess a complex interplay of characteristics, including biocompatibility, biodegradability, appropriate mechanical properties, interconnected porosity for nutrient diffusion and waste removal, and the ability to promote cell adhesion, proliferation, and differentiation [1].
While early scaffold designs were relatively simple, the field has witnessed significant advancements driven by a deeper understanding of cell-matrix interactions, biomaterial science, and fabrication technologies. The advent of 3D bioprinting has revolutionized scaffold fabrication, enabling the precise deposition of cells and biomaterials in a layer-by-layer fashion, offering unprecedented control over scaffold architecture and composition [2]. However, bioprinting is not a panacea, and limitations related to bioink development, printing resolution, and long-term mechanical stability remain. Therefore, a comprehensive understanding of alternative fabrication techniques and biomaterial options is crucial for advancing the field.
This report aims to provide an in-depth analysis of advanced scaffolding strategies, encompassing a broad perspective beyond the focus on bioprinting. We will explore the diverse range of biomaterials employed in scaffold fabrication, critically evaluate various manufacturing techniques, discuss the importance of scaffold design for specific tissue engineering applications, and address the persistent challenges in vascularization and scaffold integration. Furthermore, we will highlight emerging trends and future directions in the field, emphasizing the need for innovative approaches to overcome existing limitations and accelerate the clinical translation of tissue-engineered constructs.
Many thanks to our sponsor Esdebe who helped us prepare this research report.
2. Biomaterials for Scaffold Fabrication: A Critical Analysis
The choice of biomaterial is paramount in scaffold design, as it dictates the scaffold’s biocompatibility, biodegradability, mechanical properties, and interaction with cells. Biomaterials can be broadly classified into natural and synthetic polymers, each offering distinct advantages and disadvantages. The selection criteria should be informed by the target tissue, the desired degradation profile, and the specific cellular response required.
2.1 Natural Polymers
Natural polymers, derived from biological sources, exhibit inherent biocompatibility and biodegradability due to their structural similarity to the native ECM. Common examples include collagen, gelatin, hyaluronic acid (HA), alginate, chitosan, and silk fibroin [3].
- Collagen: The most abundant protein in the mammalian body, collagen provides excellent cell adhesion and promotes tissue regeneration. However, its mechanical strength is limited, and batch-to-batch variability can be a concern [4].
- Gelatin: A denatured form of collagen, gelatin offers improved solubility and processability compared to collagen. However, it lacks the structural integrity of collagen and may exhibit rapid degradation [5].
- Hyaluronic Acid (HA): A glycosaminoglycan (GAG) found in the ECM, HA promotes cell proliferation, migration, and angiogenesis. It is highly biocompatible but degrades rapidly in vivo, necessitating chemical modification for improved stability [6].
- Alginate: A polysaccharide derived from brown algae, alginate can be readily crosslinked with divalent cations to form hydrogels. It is biocompatible and relatively inexpensive, but its lack of cell adhesion motifs limits its application in some tissue engineering scenarios [7].
- Chitosan: A deacetylated derivative of chitin, chitosan possesses antibacterial properties and promotes wound healing. Its positively charged nature allows it to interact with negatively charged molecules, making it suitable for drug delivery applications [8].
- Silk Fibroin: A protein derived from silkworm cocoons, silk fibroin exhibits excellent mechanical strength and biocompatibility. It can be processed into various forms, including films, fibers, and hydrogels [9].
Despite their inherent advantages, natural polymers often suffer from limitations such as poor mechanical strength, rapid degradation, and potential immunogenicity. Chemical modification and crosslinking strategies are frequently employed to address these shortcomings and tailor their properties to specific applications. For example, incorporating RGD peptides into alginate scaffolds can enhance cell adhesion, while crosslinking collagen with genipin can improve its mechanical stability and degradation resistance.
2.2 Synthetic Polymers
Synthetic polymers offer greater control over material properties, such as mechanical strength, degradation rate, and pore size. They can be tailored to specific applications through careful selection of monomers and polymerization techniques. Common examples include poly(lactic acid) (PLA), poly(glycolic acid) (PGA), poly(lactic-co-glycolic acid) (PLGA), polycaprolactone (PCL), and polyethylene glycol (PEG) [10].
- PLA, PGA, and PLGA: These biodegradable polyesters are widely used in tissue engineering due to their biocompatibility and tunable degradation rates. PLA is more hydrophobic and degrades slower than PGA. PLGA copolymers offer a range of degradation rates depending on the PLA/PGA ratio [11].
- PCL: A semi-crystalline polymer with a slow degradation rate, PCL provides long-term mechanical support. It is often blended with other polymers to modulate its degradation behavior [12].
- PEG: A highly water-soluble polymer, PEG is often used to create hydrogels with excellent biocompatibility. It can be functionalized with various molecules to promote cell adhesion and growth [13].
Synthetic polymers, while offering greater control over material properties, may lack the inherent bioactivity of natural polymers. They may also elicit inflammatory responses in some cases. Surface modification techniques, such as coating with ECM proteins or peptides, can be employed to improve their biocompatibility and promote cell adhesion. Furthermore, blending synthetic polymers with natural polymers can create hybrid scaffolds that combine the advantages of both types of materials.
2.3 Beyond Traditional Polymers: Ceramics and Composites
In addition to natural and synthetic polymers, ceramics, such as hydroxyapatite (HA) and tricalcium phosphate (TCP), are frequently used in bone tissue engineering due to their osteoconductive properties. These materials promote bone cell adhesion, proliferation, and differentiation, leading to bone formation [14]. However, ceramics are brittle and lack the flexibility of polymers. Composite scaffolds, combining ceramics with polymers, can overcome these limitations, providing both mechanical strength and osteoconductivity [15].
Another emerging area is the use of bioactive glasses in scaffold fabrication. These materials release ions that stimulate bone regeneration and angiogenesis, making them attractive for bone and vascular tissue engineering applications [16].
Ultimately, the selection of the appropriate biomaterial depends on the specific requirements of the tissue engineering application. A thorough understanding of the material properties, biocompatibility, biodegradability, and interaction with cells is crucial for designing effective scaffolds.
Many thanks to our sponsor Esdebe who helped us prepare this research report.
3. Fabrication Techniques: From Traditional Methods to Bioprinting and Beyond
The fabrication technique employed to create a scaffold significantly impacts its architecture, mechanical properties, and functionality. A wide range of techniques are available, each offering distinct advantages and limitations. While 3D bioprinting has emerged as a promising technology, it is essential to consider alternative methods and their potential for specific applications.
3.1 Traditional Scaffold Fabrication Techniques
Several traditional techniques have been widely used in scaffold fabrication, including solvent casting/particulate leaching, freeze-drying, electrospinning, and gas foaming [17].
- Solvent Casting/Particulate Leaching: This method involves dissolving a polymer in a solvent, casting the solution into a mold containing porogens (e.g., salt particles, sugar crystals), and then leaching out the porogens to create a porous structure. It is a simple and versatile technique but offers limited control over pore size and distribution [18].
- Freeze-Drying: This technique involves freezing a polymer solution or suspension and then removing the solvent by sublimation under vacuum. It results in a highly porous structure with interconnected pores. The pore size and morphology can be controlled by adjusting the freezing rate and solution concentration [19].
- Electrospinning: This method uses an electric field to draw charged threads of polymer solution from a nozzle onto a collector. The resulting fibers can be collected as a non-woven mesh, forming a scaffold with high surface area and interconnected pores. Electrospinning is particularly suitable for creating scaffolds for skin and vascular tissue engineering [20].
- Gas Foaming: This technique involves saturating a polymer with a gas (e.g., carbon dioxide) under pressure and then releasing the pressure, causing the gas to expand and form pores within the polymer matrix. It offers control over pore size and distribution but may require high temperatures and pressures [21].
These traditional techniques offer simplicity and scalability but often lack the precision and control over scaffold architecture that is achievable with more advanced methods.
3.2 Advanced Fabrication Techniques: Microfluidics and Bioprinting
Advanced fabrication techniques, such as microfluidics and bioprinting, offer greater control over scaffold architecture, composition, and cell distribution [22].
- Microfluidics: This technique utilizes microchannels to precisely control fluid flow and mixing, enabling the fabrication of scaffolds with controlled pore size, shape, and interconnectivity. Microfluidic devices can be used to create hydrogel microparticles, fibers, and complex 3D structures [23]. While offering high precision, microfluidics may be limited by throughput and scalability.
- 3D Bioprinting: This technique involves the layer-by-layer deposition of cells and biomaterials to create complex 3D structures. Bioprinting offers unprecedented control over cell distribution and scaffold architecture, making it a promising technology for tissue engineering [24]. Several bioprinting techniques exist, including extrusion-based bioprinting, inkjet bioprinting, and laser-assisted bioprinting, each with its own advantages and limitations.
- Extrusion-based bioprinting: This is the most common bioprinting technique, involving the extrusion of bioinks through a nozzle. It is relatively simple and versatile but may be limited by printing resolution and cell viability due to shear stress [25].
- Inkjet bioprinting: This technique uses thermal or piezoelectric actuators to eject droplets of bioink onto a substrate. It offers high printing resolution but may be limited by the viscosity and cell density of the bioink [26].
- Laser-assisted bioprinting: This technique uses a laser to transfer cells from a ribbon onto a substrate. It offers high cell viability and precise cell placement but is more complex and expensive than other bioprinting techniques [27].
Bioprinting faces several challenges, including the development of suitable bioinks with appropriate mechanical properties and biocompatibility, the preservation of cell viability during printing, and the need for vascularization of thick, bioprinted tissues. While bioprinting holds immense potential, it is not a mature technology and requires further research and development.
3.3 Emerging Trends: Four-Dimensional (4D) Printing and Self-Assembling Scaffolds
Emerging trends in scaffold fabrication include four-dimensional (4D) printing and self-assembling scaffolds [28].
- 4D Printing: This technique involves printing scaffolds with stimuli-responsive materials that can change their shape or properties over time in response to external stimuli, such as temperature, pH, or light. This allows for the creation of scaffolds that can adapt to the changing needs of the regenerating tissue [29].
- Self-Assembling Scaffolds: This approach utilizes the inherent ability of certain molecules, such as peptides and proteins, to self-assemble into well-defined structures. Self-assembling scaffolds can provide nanoscale control over scaffold architecture and promote cell adhesion and differentiation [30].
These emerging techniques offer exciting possibilities for creating dynamic and intelligent scaffolds that can better mimic the native ECM and promote tissue regeneration.
Many thanks to our sponsor Esdebe who helped us prepare this research report.
4. Scaffold Design Considerations for Tissue Engineering Applications
The design of a scaffold should be tailored to the specific requirements of the target tissue. Factors such as porosity, pore size distribution, mechanical properties, and surface modification play crucial roles in determining the scaffold’s ability to support cell adhesion, proliferation, differentiation, and tissue formation.
4.1 Porosity and Pore Size Distribution
Porosity and pore size distribution are critical parameters that influence nutrient diffusion, waste removal, and cell infiltration within the scaffold. The optimal porosity and pore size depend on the cell type and tissue being engineered [31]. For example, bone tissue engineering requires larger pores (typically >100 μm) to allow for bone cell infiltration and vascularization, while cartilage tissue engineering may require smaller pores (typically 20-50 μm) to maintain chondrocyte phenotype [32]. Interconnected pores are essential for promoting cell migration and nutrient transport throughout the scaffold.
4.2 Mechanical Properties
The mechanical properties of the scaffold should match those of the native tissue to provide adequate structural support and prevent stress shielding. Mismatched mechanical properties can lead to implant failure and inhibit tissue regeneration [33]. For example, bone scaffolds should possess high compressive strength and stiffness, while soft tissue scaffolds, such as those for skin or cartilage, should be more compliant and elastic. The scaffold’s mechanical properties can be tailored by selecting appropriate biomaterials, adjusting the scaffold architecture, and incorporating crosslinking agents.
4.3 Surface Modification
Surface modification can be used to enhance cell adhesion, proliferation, and differentiation on the scaffold surface. Common surface modification techniques include coating with ECM proteins (e.g., collagen, fibronectin), grafting with cell adhesion peptides (e.g., RGD), and plasma treatment [34]. Surface modification can also be used to control the scaffold’s hydrophilicity and hydrophobicity, which can influence cell attachment and spreading. For example, increasing the hydrophilicity of a scaffold surface can improve cell adhesion and spreading.
4.4 Tissue-Specific Design Considerations
The design considerations for scaffolds vary depending on the tissue being engineered. For example, scaffolds for bone tissue engineering should be osteoconductive and osteoinductive, promoting bone cell adhesion, proliferation, and differentiation. Scaffolds for cartilage tissue engineering should provide a suitable microenvironment for chondrocytes to maintain their phenotype and produce cartilage matrix. Scaffolds for vascular tissue engineering should promote endothelial cell adhesion and proliferation, leading to the formation of functional blood vessels.
Many thanks to our sponsor Esdebe who helped us prepare this research report.
5. Challenges in Vascularization and Strategies for Improving Scaffold Integration
A major challenge in tissue engineering is the lack of adequate vascularization in engineered tissues. Without a functional vascular network, cells within the scaffold cannot receive sufficient nutrients and oxygen, leading to cell death and tissue necrosis. Several strategies have been developed to address this challenge, including incorporating angiogenic factors into the scaffold, creating microchannels within the scaffold, and co-culturing cells with endothelial cells [35].
5.1 Incorporating Angiogenic Factors
Angiogenic factors, such as vascular endothelial growth factor (VEGF) and basic fibroblast growth factor (bFGF), can stimulate blood vessel formation. These factors can be incorporated into the scaffold by encapsulation within microparticles, immobilization on the scaffold surface, or genetic modification of cells to produce angiogenic factors [36].
5.2 Creating Microchannels within the Scaffold
Microchannels within the scaffold can provide pathways for blood vessels to grow into the tissue. These microchannels can be created using techniques such as microfluidics, bioprinting, or sacrificial templating [37]. The size and spacing of the microchannels should be optimized to promote vascularization without compromising the mechanical properties of the scaffold.
5.3 Co-culturing Cells with Endothelial Cells
Co-culturing cells with endothelial cells can promote the formation of vascular networks within the scaffold. Endothelial cells can be seeded onto the scaffold before or during the seeding of the target cells. The interaction between the endothelial cells and the target cells can stimulate angiogenesis and improve tissue vascularization [38].
5.4 Improving Scaffold Integration
In addition to vascularization, scaffold integration with the host tissue is crucial for long-term success. Poor scaffold integration can lead to inflammation, fibrosis, and implant failure. Strategies for improving scaffold integration include surface modification to promote cell adhesion and migration, tailoring the scaffold degradation rate to match the rate of tissue formation, and incorporating growth factors to stimulate tissue regeneration [39].
Many thanks to our sponsor Esdebe who helped us prepare this research report.
6. Future Directions and Emerging Trends
The field of scaffold technology is constantly evolving, with new biomaterials, fabrication techniques, and design strategies being developed. Future research should focus on addressing the existing limitations of current scaffolds and developing innovative approaches to improve their functionality and clinical efficacy.
6.1 Personalized Scaffolds
The concept of personalized medicine is gaining increasing attention in tissue engineering. Personalized scaffolds, tailored to the specific needs of the patient, offer the potential to improve treatment outcomes. This can involve using patient-derived cells and biomaterials to create scaffolds that are biocompatible and promote tissue regeneration in the individual patient [40].
6.2 Smart Biomaterials
Smart biomaterials that respond to external stimuli, such as temperature, pH, or mechanical stress, offer the potential to create dynamic and responsive scaffolds. These materials can be used to deliver drugs, release growth factors, or change the scaffold’s mechanical properties in response to the changing needs of the regenerating tissue [41].
6.3 Advanced Characterization Techniques
Advanced characterization techniques are needed to fully understand the properties of scaffolds and their interaction with cells. This includes techniques such as micro-computed tomography (micro-CT) to assess scaffold architecture, mechanical testing to evaluate mechanical properties, and confocal microscopy to visualize cell-scaffold interactions [42].
6.4 Regulatory Considerations and Clinical Translation
The translation of engineered tissues into clinical applications requires careful consideration of regulatory issues. Scaffolds must meet stringent safety and efficacy requirements before they can be used in humans. This includes demonstrating biocompatibility, biodegradability, and the ability to promote tissue regeneration without causing adverse effects. Close collaboration between researchers, clinicians, and regulatory agencies is essential to facilitate the clinical translation of scaffold technology [43].
Many thanks to our sponsor Esdebe who helped us prepare this research report.
7. Conclusion
Scaffolds are essential components in tissue engineering and regenerative medicine, providing a structural template for cell growth and tissue formation. While 3D bioprinting has emerged as a powerful fabrication technique, it is crucial to consider alternative methods and a broad range of biomaterials to address the specific requirements of different tissue engineering applications. Careful consideration of scaffold design parameters, such as porosity, mechanical properties, and surface modification, is essential for promoting cell adhesion, proliferation, and differentiation. Overcoming the challenges in vascularization and scaffold integration is critical for the long-term success of engineered tissues. Future research should focus on developing personalized scaffolds, smart biomaterials, and advanced characterization techniques to accelerate the clinical translation of scaffold technology and realize the full potential of tissue engineering and regenerative medicine.
Many thanks to our sponsor Esdebe who helped us prepare this research report.
References
[1] Langer, R., & Tirrell, D. A. (2004). Designing materials for biology and medicine. Nature, 428(6982), 487-492.
[2] Murphy, S. V., & Atala, A. (2014). 3D bioprinting of tissues and organs. Nature Biotechnology, 32(8), 773-785.
[3] Lee, K. Y., & Mooney, D. J. (2001). Hydrogels for tissue engineering. Chemical Reviews, 101(7), 1869-1880.
[4] Pachence, J. M. (1996). Collagen-based devices for soft tissue repair. Journal of Biomedical Materials Research, 33(1), 35-40.
[5] Young, S., Wong, M., Tabata, Y., & Mikos, A. G. (2005). Gelatin as a delivery vehicle for the controlled release of bioactive molecules. Journal of Controlled Release, 109(1-3), 256-274.
[6] Necas, J., Bartosikova, L., Brauner, P., & Kolar, J. (2008). Hyaluronic acid (hyaluronan): a review. Veterinarni Medicina, 53(8), 397-411.
[7] Drury, J. L., & Mooney, D. J. (2003). Hydrogels for tissue engineering: scaffold design variables and applications. Biomaterials, 24(24), 4337-4351.
[8] Muzzarelli, R. A. A. (2011). Chitosan nanomaterials in tissue engineering. Carbohydrate Polymers, 84(1), 29-43.
[9] Kundu, B., Rajkhowa, R., Kundu, S. C., & Wang, X. (2013). Silk fibroin biomaterials for tissue regenerations. Advanced Drug Delivery Reviews, 65(4), 457-470.
[10] Nair, L. S., & Laurencin, C. T. (2007). Biodegradable polymers as biomaterials. Progress in Polymer Science, 32(8-9), 762-798.
[11] Makadia, H. K., & Siegel, S. J. (2011). Poly lactic-co-glycolic acid (PLGA) as biodegradable controlled drug delivery carrier. Polymers, 3(3), 1377-1397.
[12] Woodruff, M. A., & Hutmacher, D. W. (2010). The return of a forgotten polymer—polycaprolactone in biomedical engineering. Progress in Polymer Science, 35(10), 1217-1256.
[13] Banerjee, A., Qi, Y., Gogia, S., & Mitragotri, S. (2011). Polyethylene glycol (PEG) in skin permeation enhancement. Advanced Drug Delivery Reviews, 63(14-15), 1229-1246.
[14] Hench, L. L. (1998). Bioceramics. Journal of the American Ceramic Society, 81(7), 1705-1728.
[15] Bose, S., Varma, H. K., & Bandyopadhyay, A. (2012). Additive manufacturing of biomaterials. Progress in Materials Science, 57(8), 1178-1264.
[16] Jones, J. R. (2013). Review of bioactive glass: From Hench to hybrids. Acta Biomaterialia, 9(1), 6264-6286.
[17] O’Brien, F. J. (2011). Biomaterials & scaffolds for tissue engineering. Materials Today, 14(3), 88-95.
[18] Mikos, A. G., Temenoff, J. S. (2000). Formation of highly porous biodegradable scaffolds for tissue engineering. Electronic Journal of Biotechnology, 3(2), 114-119.
[19] Loi, F., Cordey, A., Kuhn, G., Li, M., Zysset, P., & Ito, K. (2011). Freeze-drying and crosslinking of hydrogels increases stiffness and promotes cartilage formation in vitro. Journal of Biomedical Materials Research Part A, 99(4), 620-627.
[20] Bhardwaj, N., & Kundu, S. C. (2010). Electrospinning: a fascinating fiber fabrication technique. Biotechnology Advances, 28(3), 325-347.
[21] Harris, L. D., Kim, B. S., & Mooney, D. J. (1998). Open pore biodegradable matrices formed with gas foaming. Journal of Biomedical Materials Research, 42(4), 561-567.
[22] Zhang, Y. S., & Khademhosseini, A. (2017). Advances in microfluidics for tissue engineering and stem cell biology. Integrative Biology, 9(1), 24-41.
[23] Hwang, B., Bae, W. G., Huh, D., & Lee, S. H. (2019). Microfluidics for tissue engineering and disease modeling. Lab on a Chip, 19(1), 19-33.
[24] Ozbolat, I. T., & Hospodiuk, M. (2016). Current advances and future perspectives in 3D bioprinting. Bioprinting, 5-8, 72-85.
[25] Khalil, S., Nam, K., Sun, W., & Khademhosseini, A. (2018). Multi-material bioprinting for regenerative medicine. Advanced Materials, 30(16), 1704774.
[26] Saunders, R. E., Derby, B. (2014). Inkjet printing biomaterials for tissue engineering: a review. Journal of Biomedical Materials Research Part A, 102(4), 631-648.
[27] Guillotin, B., Souquet, A., Catros, S., Duocastella, M. S., Pippenger, B., Bellon, L., … & Renaud, P. (2010). Laser-assisted bioprinting of cell/biomaterial constructs. Materials Today, 13(14), 14-22.
[28] Tibbits, S. (2014). 4D printing: multi-material shape change. Architectural Design, 84(1), 116-121.
[29] Momeni, F., Hassani, S., & Nia, H. T. (2017). 4D bioprinting. Materials Today Chemistry, 5, 19-45.
[30] Lutolf, M. P., & Hubbell, J. A. (2003). Synthesis and physicochemical characterization of end-linked poly (ethylene glycol)-co-peptide hydrogels prepared by Michael-type addition. Biomacromolecules, 4(4), 713-722.
[31] Karageorgiou, V., & Kaplan, D. (2005). Porosity of 3D biomaterial scaffolds and osteogenesis. Biomaterials, 26(27), 5474-5491.
[32] Burg, K. J. L., Porter, S., & Kellam, J. F. (2000). Biomaterial developments for bone tissue engineering. Biomaterials, 21(23), 2347-2359.
[33] Lutolf, M. P., Weber, F. E., Schmoekel, H. G., Schense, J. C., Kohler, T., Müller, R., & Hubbell, J. A. (2003). Repair of bone defects using synthetic matrices with tailored mechanical properties. Nature Biotechnology, 21(5), 513-518.
[34] Keselowsky, B. G., Collard, D. M., & Garcia, A. J. (2005). Integrin binding specificity regulates biomaterial surface-directed cell migration. Proceedings of the National Academy of Sciences, 102(17), 5953-5957.
[35] Rouwkema, J., Rivron, N. C., & van Blitterswijk, C. A. (2008). Vascularization in tissue engineering: a review. Trends in Biotechnology, 26(8), 434-441.
[36] Zisch, A. H., Lutolf, M. P., Ehrbar, M., Raeber, G. P., Rizzi, S. C., Davies, N., … & Hubbell, J. A. (2003). Cell-demanded release of VEGF from synthetic, biointeractive cell ingrowth matrices for vascularized tissue engineering. FASEB Journal, 17(15), 2260-2262.
[37] Laschke, M. W., & Menger, M. D. (2017). Mesenchymal stem cells in vascularized tissue engineering: recent advances and future perspectives. European Surgical Research, 58(1-2), 1-13.
[38] Koike, N., Tsuchiya, T., Ohshima, H., & Nakayama, K. (2004). Creation of perfused microvascular networks within engineered tissues. Biomaterials, 25(18), 3523-3531.
[39] Badylak, S. F. (2002). Xenogeneic extracellular matrix as a scaffold for tissue reconstruction. Transplantation, 74(5), 635-644.
[40] Datta, P., Baruffaldi Preisig, D., et al. (2017). Current Perspectives on 3D Bioprinting for Tissue and Organ Regeneration. Journal of Reconstructive Microsurgery, 33(07), 438-447.
[41] Hoffman, A. S. (2012). ‘Smart’ polymers in biomedical applications. European Polymer Journal, 48(4), 553-562.
[42] Herda, R., Maurer, A., & Müller, R. (2010). Advanced imaging techniques in bone tissue engineering. European Cells and Materials, 20, 125-141.
[43] Lysaght, M. J., & Hazlehurst, A. L. (2004). Tissue engineering: the regulatory landscape. Tissue Engineering, 10(3-4), 356-366.
Personalized scaffolds? Sounds incredibly bespoke! I’m now imagining tiny, scaffold-making robots, trained on my own cells and bio-data. But what happens when my cells start demanding a luxury condo scaffold with a rooftop pool? Will Esdebe sponsor *that* research, too?
That’s a hilarious and insightful point! The idea of cells dictating scaffold design is definitely a futuristic (and expensive!) scenario. Perhaps future research will focus on biodegradable pools! We appreciate Esdebe’s commitment to pushing the boundaries of what’s possible in tissue engineering, even if luxury condos are a *little* outside our scope right now.
Editor: MedTechNews.Uk
Thank you to our Sponsor Esdebe
The discussion of smart biomaterials opens exciting avenues. Could self-assembling scaffolds incorporating stimuli-responsive elements offer enhanced control over cellular microenvironments and accelerate tissue regeneration?