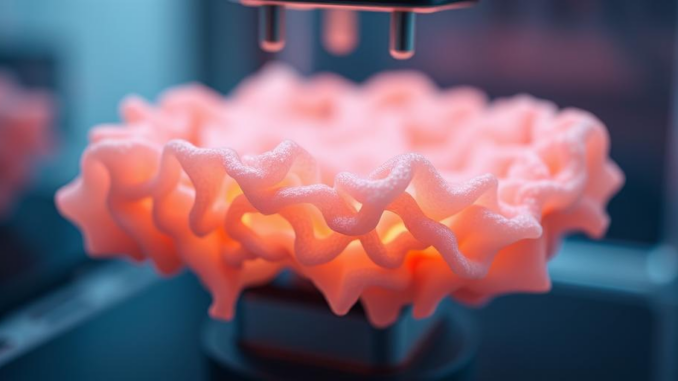
Abstract
Bioprinting has emerged as a promising technology within the realm of tissue engineering and regenerative medicine, offering the potential to fabricate complex, three-dimensional biological constructs. While initial research focused heavily on generating whole organs for transplantation, the field is rapidly evolving, expanding its scope to encompass diverse applications beyond organ replacement. This research report provides a comprehensive overview of bioprinting, examining its fundamental techniques, bioink formulations, progress in printing various tissues and organ components, and the challenges associated with scale-up and functionality. Beyond the well-trodden path of whole organ fabrication, this report explores the burgeoning applications of bioprinting in drug discovery, personalized medicine, disease modeling, and fundamental biological research. We also delve into the ethical considerations that accompany this rapidly advancing technology, emphasizing the need for responsible development and implementation. Finally, the report analyzes future directions, highlighting emerging trends and potential breakthroughs that could shape the future of bioprinting.
Many thanks to our sponsor Esdebe who helped us prepare this research report.
1. Introduction
Bioprinting, at its core, is an additive manufacturing process that utilizes cells and biomaterials to create three-dimensional tissue structures with precise control over spatial organization. Building upon the principles of 3D printing, bioprinting offers the potential to overcome limitations associated with traditional tissue engineering approaches, such as scaffold-based methods, which often struggle to replicate the intricate microenvironment of native tissues [1]. Initially conceived as a means to generate functional organs for transplantation, the field has expanded considerably. The inherent advantages of bioprinting, including the ability to deposit cells and biomaterials with high precision, create complex architectures, and customize constructs based on patient-specific data, have opened new avenues for research and clinical applications beyond whole organ fabrication.
This report aims to provide a comprehensive overview of the bioprinting landscape, moving beyond the frequently discussed topic of organ printing. We will delve into the various bioprinting techniques, bioink compositions, and tissue-specific applications, while also examining the challenges and ethical considerations surrounding this technology. Furthermore, we will explore the emerging applications of bioprinting in drug discovery, personalized medicine, and disease modeling, showcasing its potential to revolutionize various aspects of healthcare and biological research.
Many thanks to our sponsor Esdebe who helped us prepare this research report.
2. Bioprinting Techniques: A Comparative Analysis
Several bioprinting techniques have been developed, each with its own advantages and limitations. Understanding the nuances of these techniques is crucial for selecting the most appropriate method for a specific application. The three primary bioprinting approaches are extrusion-based, inkjet-based, and laser-induced forward transfer (LIFT) bioprinting.
2.1. Extrusion-Based Bioprinting
Extrusion-based bioprinting is arguably the most widely used technique due to its simplicity, versatility, and relatively low cost [2]. This method involves dispensing bioinks through a nozzle or micro-capillary using pneumatic pressure, mechanical displacement (e.g., screw-based or piston-based), or other driving forces. The bioink is deposited layer-by-layer onto a substrate, creating a three-dimensional structure. Extrusion-based bioprinting is suitable for a wide range of bioink viscosities and cell densities, making it compatible with various cell types and biomaterials. However, the resolution of extrusion-based bioprinting is generally lower compared to other techniques, and the shear stress exerted on cells during extrusion can potentially affect cell viability [3]. Several variations exist, including pressure-assisted, microvalve-based, and sacrificial printing, to improve resolution and control over deposition.
2.2. Inkjet-Based Bioprinting
Inkjet-based bioprinting, inspired by conventional inkjet printing, utilizes thermal or piezoelectric actuators to eject small droplets of bioink onto a substrate [4]. This technique offers high resolution and speed, making it suitable for creating complex patterns and structures. However, inkjet-based bioprinting is limited to low-viscosity bioinks and relatively low cell densities, which can restrict its applicability for certain cell types and tissue engineering applications. Furthermore, the thermal or mechanical stress associated with droplet ejection can potentially impact cell viability. Continuous inkjet and drop-on-demand (DOD) inkjet are two main types of inkjet bioprinting systems, each with its own advantages regarding speed and material usage.
2.3. Laser-Induced Forward Transfer (LIFT) Bioprinting
LIFT bioprinting employs a laser to transfer biomaterials from a ribbon coated with bioink onto a substrate [5]. A pulsed laser beam is focused onto the ribbon, causing localized vaporization and transferring the bioink to the receiving substrate. LIFT bioprinting offers high resolution and precise control over cell deposition, minimizing cell damage due to the non-contact nature of the process. However, LIFT bioprinting is more complex and expensive compared to extrusion-based and inkjet-based methods, and it is typically limited to printing thin layers of cells. Furthermore, the type of materials that can be used is limited. The efficiency of transfer, characterized by the size and shape of the transferred material, must be carefully controlled.
2.4. Emerging Bioprinting Techniques
Beyond the three main techniques, several emerging bioprinting methods are gaining traction. These include volumetric bioprinting, which uses light to solidify entire volumes of bioink simultaneously, and bioprinting within microfluidic devices, which allows for precise control over the microenvironment surrounding the printed cells [6, 7]. These advanced techniques aim to overcome the limitations of conventional bioprinting methods, offering improved resolution, throughput, and control over the printed constructs.
Many thanks to our sponsor Esdebe who helped us prepare this research report.
3. Bioinks: The Building Blocks of Bioprinted Tissues
Bioinks are the essential materials used in bioprinting, serving as the vehicle for delivering cells and providing structural support to the printed constructs. The ideal bioink should possess several key characteristics, including biocompatibility, biodegradability, printability, and the ability to promote cell adhesion, proliferation, and differentiation [8]. The selection of the appropriate bioink is crucial for achieving successful bioprinting outcomes. The choice of bioink is highly dependent on the specific cell type, application, and bioprinting technique employed.
3.1. Hydrogels
Hydrogels are three-dimensional networks of cross-linked polymers that can absorb and retain large amounts of water. They are widely used as bioinks due to their biocompatibility, tunable mechanical properties, and ability to mimic the extracellular matrix (ECM) [9]. Common hydrogels used in bioprinting include natural polymers such as collagen, gelatin, alginate, hyaluronic acid, and chitosan, as well as synthetic polymers such as polyethylene glycol (PEG) and poly(lactic-co-glycolic acid) (PLGA). Natural hydrogels offer excellent biocompatibility and cell adhesion properties but may exhibit batch-to-batch variability and limited mechanical strength. Synthetic hydrogels provide better control over mechanical properties and degradation rates but may lack the inherent bioactivity of natural materials. Blending natural and synthetic hydrogels is a common approach to combine the advantages of both types of materials.
3.2. Cell Aggregates
Cell aggregates, also known as spheroids or microtissues, are three-dimensional clusters of cells that exhibit enhanced cell-cell interactions and ECM deposition compared to individual cells [10]. Cell aggregates can be directly bioprinted without the need for a supporting matrix, allowing for the creation of highly cellular constructs. However, maintaining the integrity of cell aggregates during printing and ensuring their fusion after deposition can be challenging. The shape and size uniformity of the initial spheroids is also a critical factor.
3.3. Decellularized Extracellular Matrix (dECM)
dECM is derived from native tissues by removing all cellular components while preserving the structural and compositional integrity of the ECM [11]. dECM bioinks offer excellent biocompatibility and bioactivity, providing cells with the natural cues necessary for tissue regeneration. However, obtaining sufficient quantities of dECM can be challenging, and the mechanical properties of dECM bioinks may need to be adjusted to meet the requirements of bioprinting.
3.4. Composite Bioinks
Composite bioinks combine multiple materials to create bioinks with tailored properties. For example, a composite bioink may consist of a hydrogel matrix reinforced with nanoparticles or microfibers to improve mechanical strength and printability [12]. The addition of growth factors or other bioactive molecules to bioinks can further enhance cell behavior and tissue regeneration. The design of composite bioinks requires careful consideration of the interactions between the different components and their impact on cell viability and function.
Many thanks to our sponsor Esdebe who helped us prepare this research report.
4. Bioprinting Applications: Beyond Organ Fabrication
While the prospect of printing whole organs for transplantation has captured significant attention, bioprinting has a much broader range of potential applications that are rapidly gaining momentum. These include the creation of functional tissues for regenerative medicine, the development of in vitro models for drug discovery and disease modeling, and the fabrication of personalized medical devices.
4.1. Skin Bioprinting
Skin bioprinting has emerged as a promising approach for treating severe burns, chronic wounds, and other skin defects [13]. Bioprinted skin grafts can be generated using patient-derived cells, reducing the risk of rejection and promoting faster healing. Several companies are currently developing bioprinted skin products for clinical use, and early clinical trials have shown promising results. The ability to bioprint complex skin structures, including multiple layers of cells and vascular networks, is crucial for achieving functional skin regeneration.
4.2. Bone and Cartilage Bioprinting
Bone and cartilage defects are common clinical problems that can be challenging to treat with conventional methods. Bioprinting offers the potential to create customized bone and cartilage implants that can promote tissue regeneration and restore function [14]. Bioprinted bone constructs can be generated using osteoblasts and bone-mimicking biomaterials, while bioprinted cartilage constructs can be created using chondrocytes and cartilage-specific ECM components. The mechanical properties and degradation rates of the bioprinted scaffolds must be carefully tailored to match the requirements of the specific bone or cartilage defect.
4.3. Vascular Bioprinting
The creation of functional vascular networks is essential for the survival and integration of bioprinted tissues [15]. Bioprinting techniques can be used to create complex vascular networks that can deliver nutrients and oxygen to cells within the printed constructs. Several approaches have been developed for vascular bioprinting, including the use of sacrificial materials, self-assembling peptides, and microfluidic devices. The integration of bioprinted vascular networks with existing blood vessels in the host tissue is a critical challenge that needs to be addressed for successful tissue regeneration.
4.4. Drug Discovery and Disease Modeling
Bioprinting provides a powerful platform for creating in vitro models that mimic the complexity of human tissues and organs [16]. These models can be used for drug screening, toxicity testing, and disease modeling, reducing the reliance on animal models and accelerating the drug development process. Bioprinted tumor models, for example, can be used to study cancer cell behavior and test the efficacy of anticancer drugs. The ability to create patient-specific bioprinted models opens new avenues for personalized medicine, allowing clinicians to tailor treatment strategies based on individual patient responses.
4.5. Personalized Medicine
Bioprinting enables the creation of patient-specific implants and tissues, tailored to an individual’s unique anatomy and medical needs [17]. This personalized approach can improve the outcomes of surgical procedures, reduce the risk of rejection, and enhance the overall quality of life for patients. The use of patient-derived cells in bioprinting ensures biocompatibility and minimizes the immune response. However, the cost and time associated with generating patient-specific bioprinted constructs remain significant challenges.
Many thanks to our sponsor Esdebe who helped us prepare this research report.
5. Challenges and Future Directions
Despite the significant progress made in bioprinting, several challenges remain that need to be addressed before this technology can be widely implemented in clinical practice.
5.1. Scale-Up and Manufacturing
Scaling up the production of bioprinted tissues and organs is a major hurdle [18]. Current bioprinting techniques are often slow and labor-intensive, limiting their applicability for large-scale manufacturing. Developing automated bioprinting systems and optimizing bioink formulations are crucial for increasing throughput and reducing production costs. Standardization of bioprinting processes and quality control measures are also essential for ensuring the consistency and reproducibility of bioprinted products.
5.2. Vascularization and Perfusion
Creating functional vascular networks that can adequately perfuse bioprinted tissues is a major challenge [19]. Larger and more complex bioprinted constructs require sophisticated vascularization strategies to ensure cell survival and integration. Developing methods for creating hierarchical vascular networks and connecting them to existing blood vessels in the host tissue is a critical area of research.
5.3. Bioreactor Development and Maturation
Bioprinted tissues often require maturation in bioreactors to develop their full functionality [20]. Bioreactors provide a controlled environment that can mimic the physiological conditions of the native tissue, promoting cell differentiation and ECM deposition. Developing bioreactors that can provide appropriate mechanical stimulation, nutrient supply, and waste removal is essential for achieving functional tissue maturation.
5.4. Regulatory and Ethical Considerations
Bioprinting raises several regulatory and ethical considerations that need to be carefully addressed [21]. The safety and efficacy of bioprinted products must be thoroughly evaluated before they can be approved for clinical use. Issues such as intellectual property, data privacy, and the potential for misuse of bioprinting technology need to be addressed through appropriate regulations and guidelines. Public engagement and education are also crucial for ensuring the responsible development and implementation of bioprinting.
5.5. Future Directions
The future of bioprinting holds immense potential. Emerging trends include the development of multi-material bioprinting, which allows for the creation of complex tissues with multiple cell types and biomaterials; the integration of artificial intelligence (AI) and machine learning (ML) to optimize bioprinting parameters and predict tissue behavior; and the use of CRISPR-Cas9 gene editing to enhance the functionality of bioprinted cells [22, 23, 24]. These advancements will further expand the capabilities of bioprinting and pave the way for new applications in regenerative medicine, drug discovery, and personalized healthcare. The convergence of bioprinting with other technologies, such as microfluidics, nanotechnology, and robotics, will further accelerate the development of advanced tissue engineering solutions.
Many thanks to our sponsor Esdebe who helped us prepare this research report.
6. Conclusion
Bioprinting has evolved from a nascent technology focused primarily on organ fabrication to a versatile platform with diverse applications in tissue engineering, regenerative medicine, drug discovery, and personalized medicine. While the goal of printing whole organs for transplantation remains a long-term aspiration, the immediate impact of bioprinting is being felt in areas such as skin regeneration, bone and cartilage repair, and the development of in vitro models for disease research. The continuous advancements in bioprinting techniques, bioink formulations, and bioreactor technology are driving the field forward, overcoming existing challenges and expanding the scope of its applications. As bioprinting matures, it has the potential to revolutionize healthcare and improve the lives of millions of people. However, responsible development, careful regulation, and ongoing ethical considerations are crucial to ensure that this powerful technology is used for the benefit of society.
Many thanks to our sponsor Esdebe who helped us prepare this research report.
References
[1] Murphy, S. V., & Atala, A. (2014). 3D bioprinting of tissues and organs. Nature Biotechnology, 32(8), 773-785.
[2] Ozbolat, I. T., & Hospodiuk, M. (2016). Current approaches and future perspectives in 3D bioprinting. Biomaterials, 76, 321-343.
[3] Blaeser, A., Duarte Campos, D. F., & Zenobi-Wong, M. (2016). Controlling the cellular microenvironment in bioprinting. Advanced Healthcare Materials, 5(3), 336-362.
[4] Saunders, R. E., Derby, B. (2014). Inkjet printing biomaterials for tissue engineering. Rep. Prog. Phys. 77, 036601.
[5] Barron, J. A., Wu, P., Ladewig, K., Ovsianikov, A., & Forgacs, G. (2017). Bioprinting for fabrication of biological systems. Advanced Healthcare Materials, 6(1), 1600658.
[6] Laronda, M. M., Rutz, A. L., Xiao, S., Duncan, F. E., Roth, E. W., Woodruff, T. K., & Shah, R. N. (2017). A bioprosthetic ovary created using 3D printing restores ovarian function in sterilized mice. Nature Communications, 8(1), 15261.
[7] Kolesky, D. B., Truby, R. L., Gladman, A. S., Busbee, T. A., Homan, K. A., & Lewis, J. A. (2014). 3D bioprinting of vascularized, heterogeneous cell-laden tissue constructs. Advanced Materials, 26(19), 3124-3130.
[8] Gungor-Ozkerim, P. S., Inci, I., Zhang, Y. S., Khademhosseini, A., & Dokmeci, M. R. (2018). Bioprinting: Fabrication of tissues and organs. Trends in Biotechnology, 36(3), 316-334.
[9] Lee, K. Y., & Mooney, D. J. (2012). Alginate: Properties and biomedical applications. Progress in Polymer Science, 37(1), 106-126.
[10] Barthes, J., Özçelik, B., Hindie, E., Ndreu-Halili, A., Hasan, A., & Vrana, N. E. (2014). Cell microenvironment engineering and monitoring for tissue engineering and regenerative medicine: The convergence of advanced materials, fabrication and imaging technologies. Advanced Healthcare Materials, 3(5), 609-635.
[11] Badylak, S. F., Gilbert, T. W. (2008). Immune response to biologic scaffold materials. Semin Immunol 20, 109–116.
[12] Chimene, D., Lennox, K. K., Garcia, A. J., & Guldberg, R. E. (2015). Advanced bioink formulations for regenerative medicine. Advanced Materials, 27(44), 7263-7282.
[13] Cubo, E., Garcia, M., del Cañizo, J. F., Talavera, A., & Espinosa, J. (2017). Clinical application of autologous cell spray for treatment of severe burns: A controlled clinical trial. Burns, 43(8), 1607-1614.
[14] Bose, S., Vahabzadeh, S., & Bandyopadhyay, A. (2013). Bone tissue engineering using 3D printing. Materials Today, 16(12), 496-504.
[15] Laschke, M. W., & Menger, M. D. (2017). In vivo assessment of skin and soft tissue microcirculation: techniques, applications, and future directions. European Surgical Research, 58(5-6), 359-373.
[16] Ingber, D. E. (2008). Mechanobiology and diseases of mechanotransduction. Annals of the New York Academy of Sciences, 1123(1), 1-17.
[17] Khademhosseini, A., & Langer, R. (2016). Personalized medicine in tissue engineering. JOM, 68(3), 713-721.
[18] Mandrycky, C., Ng, K. W., & Yeong, W. Y. (2016). Additive manufacturing of tissues and organs: Progress, challenges, and future directions. Biotechnology Advances, 34(4), 422-434.
[19] Seifu, D. G., Purnama, A., Mequanint, K., & Mantovani, D. (2013). Opportunities and challenges of applying biomaterials in tissue engineering and regenerative medicine: A Canadian perspective. Journal of Biomaterials and Tissue Engineering, 3(3), 205-224.
[20] Martin, I., Wendt, D., & Heberer, M. (2004). The role of bioreactors in tissue engineering. Trends in Biotechnology, 22(2), 80-86.
[21] DeGrazia, J., & Vodovotz, Y. (2015). Bioprinting regulatory considerations. Biofabrication, 7(3), 030201.
[22] Lee, J. M., Choi, Y. J., Kim, J. Y., & Kim, J. Y. (2021). 3D bioprinting for tissue engineering: Current progress and future perspectives. International Journal of Bioprinting, 7(1).
[23] Vijayavenkataraman, S., Yan, H., Lu, W. F., Wang, K., Nguyen, N. T., & Qin, L. (2021). Artificial intelligence in bioprinting. Bioprinting, 23, e00153.
[24] Park, S. A., Hong, S., Shim, H. J., Park, J. H., Yoon, J. W., Lee, D. H., … & Cho, D. W. (2023). CRISPR/Cas9‐Mediated Genome Editing for Enhanced Bioprinting of Functional Tissues. Advanced Healthcare Materials, 12(27), 2300480.
So, we’re “beyond organ fabrication” now? Does that mean I can’t get a spare liver printed up when my late-night karaoke habit catches up with me? Just curious.
That’s a great question! While full organ replacement is still a significant hurdle, bioprinting is making incredible strides in creating functional tissue patches and models for drug testing. Think of it less as a spare parts store *right now*, and more about repairing and understanding organ function better! Exciting times ahead!
Editor: MedTechNews.Uk
Thank you to our Sponsor Esdebe
So, if bioprinting can create personalized medicine, does that mean I can finally get a 3D-printed clone… purely for, uh, *research* purposes, of course? Asking for a friend…who is also me.
That’s a *very* interesting research proposal! While a full clone is still in the realm of science fiction for now, bioprinting is making incredible strides in creating personalized medicine. This may result in tailor made solutions, with materials designed for your body in mind, so perhaps personalised materials is something to look forward to!
Editor: MedTechNews.Uk
Thank you to our Sponsor Esdebe
The discussion on bioink formulations is particularly interesting. The ability to tailor composite bioinks with specific properties opens exciting possibilities for creating more complex and functional tissue constructs. How might these advancements impact long-term implant success?